Fast and slow γ rhythms are intrinsically and independently generated in the subiculum
- PMID: 21865453
- PMCID: PMC6623234
- DOI: 10.1523/JNEUROSCI.1370-11.2011
Fast and slow γ rhythms are intrinsically and independently generated in the subiculum
Abstract
Gamma rhythms are essential for memory encoding and retrieval. Despite extensive study of these rhythms in the entorhinal cortex, dentate gyrus, CA3, and CA1, almost nothing is known regarding their generation and organization in the structure delivering the most prominent hippocampal output: the subiculum. Here we show using a complete rat hippocampal preparation in vitro that the subiculum intrinsically and independently generates spontaneous slow (25-50 Hz) and fast (100-150 Hz) gamma rhythms during the rising phase and peak of persistent subicular theta rhythms. These two gamma frequencies are phase modulated by theta rhythms without any form of afferent input from the entorhinal cortex or CA1. Subicular principal cells and interneurons phase lock to both fast and slow gamma, and single cells are independently phase modulated by each form of gamma rhythm, enabling selective participation in neural synchrony at both gamma frequencies at different times. Fast GABAergic inhibition is required for the generation of fast gamma, whereas slow gamma is generated by excitatory and inhibitory mechanisms. In addition, the transverse subicular axis exhibits gamma rhythm topography with faster gamma coupling arising in the distal subiculum region. The subiculum therefore possesses a unique intrinsic circuit organization that can autonomously regulate the timing and topography of hippocampal output synchronization. These results suggest the subiculum is a third spontaneous gamma generator in the hippocampal formation (in addition to CA3 and the entorhinal cortex), and these gamma rhythms likely play an active role in mediating the flow of information between the hippocampus and multiple cortical and subcortical brain regions.
Figures
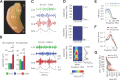
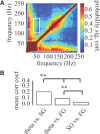
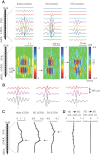
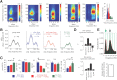
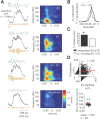
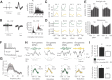
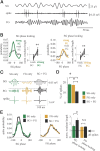
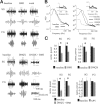
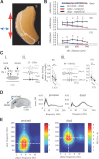
Similar articles
-
Limbic gamma rhythms. II. Synaptic and intrinsic mechanisms underlying spike doublets in oscillating subicular neurons.J Neurophysiol. 1998 Jul;80(1):162-71. doi: 10.1152/jn.1998.80.1.162. J Neurophysiol. 1998. PMID: 9658038
-
Theta phase segregation of input-specific gamma patterns in entorhinal-hippocampal networks.Neuron. 2014 Oct 22;84(2):470-85. doi: 10.1016/j.neuron.2014.08.051. Epub 2014 Sep 25. Neuron. 2014. PMID: 25263753 Free PMC article.
-
Reversal of theta rhythm flow through intact hippocampal circuits.Nat Neurosci. 2014 Oct;17(10):1362-70. doi: 10.1038/nn.3803. Epub 2014 Aug 31. Nat Neurosci. 2014. PMID: 25174002
-
Hippocampus as comparator: role of the two input and two output systems of the hippocampus in selection and registration of information.Hippocampus. 2001;11(5):578-98. doi: 10.1002/hipo.1073. Hippocampus. 2001. PMID: 11732710 Review.
-
Theta-gamma coupling in the entorhinal-hippocampal system.Curr Opin Neurobiol. 2015 Apr;31:45-50. doi: 10.1016/j.conb.2014.08.001. Epub 2014 Aug 27. Curr Opin Neurobiol. 2015. PMID: 25168855 Free PMC article. Review.
Cited by
-
Mechanisms of gamma oscillations.Annu Rev Neurosci. 2012;35:203-25. doi: 10.1146/annurev-neuro-062111-150444. Epub 2012 Mar 20. Annu Rev Neurosci. 2012. PMID: 22443509 Free PMC article. Review.
-
Early classification of Alzheimer's disease phenotype based on hippocampal electrophysiology in the TgF344-AD rat model.iScience. 2023 Jul 22;26(8):107454. doi: 10.1016/j.isci.2023.107454. eCollection 2023 Aug 18. iScience. 2023. PMID: 37599835 Free PMC article.
-
Precocious Alterations of Brain Oscillatory Activity in Alzheimer's Disease: A Window of Opportunity for Early Diagnosis and Treatment.Front Cell Neurosci. 2015 Dec 21;9:491. doi: 10.3389/fncel.2015.00491. eCollection 2015. Front Cell Neurosci. 2015. PMID: 26733816 Free PMC article. Review.
-
Tuning in the Hippocampal Theta Band In Vitro: Methodologies for Recording from the Isolated Rodent Septohippocampal Circuit.J Vis Exp. 2017 Aug 2;(126):55851. doi: 10.3791/55851. J Vis Exp. 2017. PMID: 28809843 Free PMC article.
-
Running speed and REM sleep control two distinct modes of rapid interhemispheric communication.Cell Rep. 2022 Jul 5;40(1):111028. doi: 10.1016/j.celrep.2022.111028. Cell Rep. 2022. PMID: 35793619 Free PMC article.
References
-
- Berens P. CircStat: a Matlab toolbox for circular statistics. J Statistical Software. 2009;31:1–21.
Publication types
MeSH terms
Grants and funding
LinkOut - more resources
Full Text Sources
Miscellaneous