A guide to analysis of cardiac phenotypes in the zebrafish embryo
- PMID: 21550443
- PMCID: PMC3292854
- DOI: 10.1016/B978-0-12-387036-0.00007-4
A guide to analysis of cardiac phenotypes in the zebrafish embryo
Abstract
The zebrafish is an ideal model organism for investigating the molecular mechanisms underlying cardiogenesis, due to the powerful combination of optical access to the embryonic heart and plentiful opportunities for genetic analysis. A continually increasing number of studies are uncovering mutations, morpholinos, and small molecules that cause striking cardiac defects and disrupt blood circulation in the zebrafish embryo. Such defects can result from a wide variety of origins including defects in the specification or differentiation of cardiac progenitor cells; errors in the morphogenesis of the heart tube, the cardiac chambers, or the atrioventricular canal or problems with establishing proper cardiac function. An extensive arsenal of techniques is available to distinguish between these possibilities and thereby decipher the roots of cardiac defects. In this chapter, we provide a guide to the experimental strategies that are particularly effective for the characterization of cardiac phenotypes in the zebrafish embryo.
Copyright © 2011 Elsevier Inc. All rights reserved.
Figures
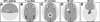
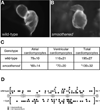
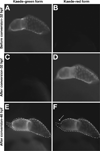
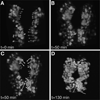
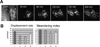
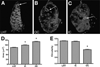
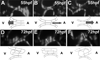
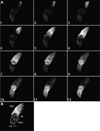
Similar articles
-
Strategies for analyzing cardiac phenotypes in the zebrafish embryo.Methods Cell Biol. 2016;134:335-68. doi: 10.1016/bs.mcb.2016.03.002. Epub 2016 Apr 4. Methods Cell Biol. 2016. PMID: 27312497 Free PMC article.
-
The atypical Rho GTPase, RhoU, regulates cell-adhesion molecules during cardiac morphogenesis.Dev Biol. 2014 May 15;389(2):182-91. doi: 10.1016/j.ydbio.2014.02.014. Epub 2014 Mar 7. Dev Biol. 2014. PMID: 24607366 Free PMC article.
-
Fluid forces shape the embryonic heart: Insights from zebrafish.Curr Top Dev Biol. 2019;132:395-416. doi: 10.1016/bs.ctdb.2018.12.009. Epub 2019 Jan 2. Curr Top Dev Biol. 2019. PMID: 30797515 Free PMC article. Review.
-
Uncovering the molecular and cellular mechanisms of heart development using the zebrafish.Annu Rev Genet. 2012;46:397-418. doi: 10.1146/annurev-genet-110711-155646. Epub 2012 Sep 4. Annu Rev Genet. 2012. PMID: 22974299 Free PMC article. Review.
-
Illuminating cardiac development: Advances in imaging add new dimensions to the utility of zebrafish genetics.Semin Cell Dev Biol. 2007 Feb;18(1):27-35. doi: 10.1016/j.semcdb.2006.12.010. Epub 2006 Dec 27. Semin Cell Dev Biol. 2007. PMID: 17241801 Free PMC article. Review.
Cited by
-
Natterin-like depletion by CRISPR/Cas9 impairs zebrafish (Danio rerio) embryonic development.BMC Genomics. 2022 Feb 12;23(1):123. doi: 10.1186/s12864-022-08369-z. BMC Genomics. 2022. PMID: 35151271 Free PMC article.
-
Haemodynamic dependence of mechano-genetic evolution of the cardiovascular system in Japanese medaka.J R Soc Interface. 2021 Oct;18(183):20210752. doi: 10.1098/rsif.2021.0752. Epub 2021 Oct 27. J R Soc Interface. 2021. PMID: 34699728 Free PMC article.
-
Scale space detector for analyzing spatiotemporal ventricular contractility and nuclear morphogenesis in zebrafish.iScience. 2022 Aug 5;25(9):104876. doi: 10.1016/j.isci.2022.104876. eCollection 2022 Sep 16. iScience. 2022. PMID: 36034231 Free PMC article.
-
Biomechanics of early cardiac development.Biomech Model Mechanobiol. 2012 Nov;11(8):1187-204. doi: 10.1007/s10237-012-0414-7. Epub 2012 Jul 4. Biomech Model Mechanobiol. 2012. PMID: 22760547 Free PMC article.
-
Block the function of nonmuscle myosin II by blebbistatin induces zebrafish embryo cardia bifida.In Vitro Cell Dev Biol Anim. 2015 Mar;51(3):211-7. doi: 10.1007/s11626-014-9836-0. Epub 2014 Nov 18. In Vitro Cell Dev Biol Anim. 2015. PMID: 25403653
References
-
- Alexander J, Stainier DY, Yelon D. Screening mosaic F1 females for mutations affecting zebrafish heart induction and patterning. Dev. Genet. 1998;22:288–299. - PubMed
-
- Alexander J, Rothenberg M, Henry GL, Stainier DY. casanova plays an early and essential role in endoderm formation in zebrafish. Dev. Biol. 1999;215:343–357. - PubMed
Publication types
MeSH terms
Grants and funding
LinkOut - more resources
Full Text Sources