Superoxide dismutases: role in redox signaling, vascular function, and diseases
- PMID: 21473702
- PMCID: PMC3151424
- DOI: 10.1089/ars.2011.3999
Superoxide dismutases: role in redox signaling, vascular function, and diseases
Abstract
Excessive reactive oxygen species Revised abstract, especially superoxide anion (O₂•-), play important roles in the pathogenesis of many cardiovascular diseases, including hypertension and atherosclerosis. Superoxide dismutases (SODs) are the major antioxidant defense systems against (O₂•-), which consist of three isoforms of SOD in mammals: the cytoplasmic Cu/ZnSOD (SOD1), the mitochondrial MnSOD (SOD2), and the extracellular Cu/ZnSOD (SOD3), all of which require catalytic metal (Cu or Mn) for their activation. Recent evidence suggests that in each subcellular location, SODs catalyze the conversion of (O₂•-), H2O2, which may participate in cell signaling. In addition, SODs play a critical role in inhibiting oxidative inactivation of nitric oxide, thereby preventing peroxynitrite formation and endothelial and mitochondrial dysfunction. The importance of each SOD isoform is further illustrated by studies from the use of genetically altered mice and viral-mediated gene transfer. Given the essential role of SODs in cardiovascular disease, the concept of antioxidant therapies, that is, reinforcement of endogenous antioxidant defenses to more effectively protect against oxidative stress, is of substantial interest. However, the clinical evidence remains controversial. In this review, we will update the role of each SOD in vascular biologies, physiologies, and pathophysiologies such as atherosclerosis, hypertension, and angiogenesis. Because of the importance of metal cofactors in the activity of SODs, we will also discuss how each SOD obtains catalytic metal in the active sites. Finally, we will discuss the development of future SOD-dependent therapeutic strategies.
Figures
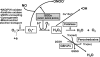
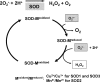
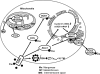
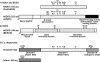
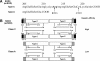
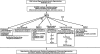
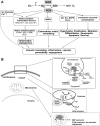
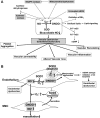
Similar articles
-
Vascular protection: superoxide dismutase isoforms in the vessel wall.Arterioscler Thromb Vasc Biol. 2004 Aug;24(8):1367-73. doi: 10.1161/01.ATV.0000133604.20182.cf. Epub 2004 May 27. Arterioscler Thromb Vasc Biol. 2004. PMID: 15166009 Review.
-
Comparative analysis of cyanobacterial superoxide dismutases to discriminate canonical forms.BMC Genomics. 2007 Nov 27;8:435. doi: 10.1186/1471-2164-8-435. BMC Genomics. 2007. PMID: 18042279 Free PMC article.
-
Zymographic Method for Distinguishing Different Classes of Superoxide Dismutases in Plants.Methods Mol Biol. 2017;1631:221-227. doi: 10.1007/978-1-4939-7136-7_13. Methods Mol Biol. 2017. PMID: 28735400
-
Redox Regulation of the Superoxide Dismutases SOD3 and SOD2 in the Pulmonary Circulation.Adv Exp Med Biol. 2017;967:57-70. doi: 10.1007/978-3-319-63245-2_5. Adv Exp Med Biol. 2017. PMID: 29047081
-
Expanding roles of superoxide dismutases in cell regulation and cancer.Drug Discov Today. 2016 Jan;21(1):143-149. doi: 10.1016/j.drudis.2015.10.001. Epub 2015 Oct 19. Drug Discov Today. 2016. PMID: 26475962 Free PMC article. Review.
Cited by
-
The impact of reactive oxygen species in the development of cardiometabolic disorders: a review.Lipids Health Dis. 2021 Feb 27;20(1):23. doi: 10.1186/s12944-021-01435-7. Lipids Health Dis. 2021. PMID: 33639960 Free PMC article. Review.
-
TaRac6 Is a Potential Susceptibility Factor by Regulating the ROS Burst Negatively in the Wheat-Puccinia striiformis f. sp. tritici Interaction.Front Plant Sci. 2020 Jun 30;11:716. doi: 10.3389/fpls.2020.00716. eCollection 2020. Front Plant Sci. 2020. PMID: 32695124 Free PMC article.
-
Pro-oxidant and antioxidant responses in the liver and kidney of wild Goodea gracilis and their relation with halomethanes bioactivation.Fish Physiol Biochem. 2013 Dec;39(6):1603-17. doi: 10.1007/s10695-013-9812-8. Epub 2013 Jun 5. Fish Physiol Biochem. 2013. PMID: 23737147
-
The role of Tyr34 in proton-coupled electron transfer of human manganese superoxide dismutase.Res Sq [Preprint]. 2024 Jun 11:rs.3.rs-4494128. doi: 10.21203/rs.3.rs-4494128/v1. Res Sq. 2024. PMID: 38946943 Free PMC article. Preprint.
-
Reactive oxygen species-reducing strategies improve pulmonary arterial responses to nitric oxide in piglets with chronic hypoxia-induced pulmonary hypertension.Antioxid Redox Signal. 2013 May 10;18(14):1727-38. doi: 10.1089/ars.2012.4823. Epub 2013 Jan 29. Antioxid Redox Signal. 2013. PMID: 23244497 Free PMC article.
References
-
- Abid MR. Tsai JC. Spokes KC. Deshpande SS. Irani K. Aird WC. Vascular endothelial growth factor induces manganese-superoxide dismutase expression in endothelial cells by a Rac1-regulated NADPH oxidase-dependent mechanism. FASEB J. 2001;15:2548–2550. - PubMed
-
- Abreu IA. Cabelli DE. Superoxide dismutases-a review of the metal-associated mechanistic variations. Biochim Biophys Acta. 2010;1804:263–274. - PubMed
-
- Adachi T. Yamazaki N. Tasaki H. Toyokawa T. Yamashita K. Hirano K. Changes in the heparin affinity of extracellular-superoxide dismutase in patients with coronary artery atherosclerosis. Biol Pharm Bull. 1998;21:1090–1093. - PubMed
-
- Agrawal RS. Muangman S. Layne MD. Melo L. Perrella MA. Lee RT. Zhang L. Lopez-Ilasaca M. Dzau VJ. Pre-emptive gene therapy using recombinant adeno-associated virus delivery of extracellular superoxide dismutase protects heart against ischemic reperfusion injury, improves ventricular function and prolongs survival. Gene Ther. 2004;11:962–969. - PubMed
-
- Alvarez B. Demicheli V. Duran R. Trujillo M. Cervenansky C. Freeman BA. Radi R. Inactivation of human Cu,Zn superoxide dismutase by peroxynitrite and formation of histidinyl radical. Free Radic Biol Med. 2004;37:813–822. - PubMed
Publication types
MeSH terms
Substances
Grants and funding
LinkOut - more resources
Full Text Sources
Other Literature Sources
Miscellaneous