Multifaceted sequence-dependent and -independent roles for reovirus FAST protein cytoplasmic tails in fusion pore formation and syncytiogenesis
- PMID: 19759162
- PMCID: PMC2786715
- DOI: 10.1128/JVI.01667-09
Multifaceted sequence-dependent and -independent roles for reovirus FAST protein cytoplasmic tails in fusion pore formation and syncytiogenesis
Abstract
Fusogenic reoviruses utilize the FAST proteins, a novel family of nonstructural viral membrane fusion proteins, to induce cell-cell fusion and syncytium formation. Unlike the paradigmatic enveloped virus fusion proteins, the FAST proteins position the majority of their mass within and internal to the membrane in which they reside, resulting in extended C-terminal cytoplasmic tails (CTs). Using tail truncations, we demonstrate that the last 8 residues of the 36-residue CT of the avian reovirus p10 FAST protein and the last 20 residues of the 68-residue CT of the reptilian reovirus p14 FAST protein enhance, but are not required for, pore expansion and syncytium formation. Further truncations indicate that the membrane-distal 12 residues of the p10 and 47 residues of the p14 CTs are essential for pore formation and that a residual tail of 21 to 24 residues that includes a conserved, membrane-proximal polybasic region present in all FAST proteins is insufficient to maintain FAST protein fusion activity. Unexpectedly, a reextension of the tail-truncated, nonfusogenic p10 and p14 constructs with scrambled versions of the deleted sequences restored pore formation and syncytiogenesis, while reextensions with heterologous sequences partially restored pore formation but failed to rescue syncytiogenesis. The membrane-distal regions of the FAST protein CTs therefore exert multiple effects on the membrane fusion reaction, serving in both sequence-dependent and sequence-independent manners as positive effectors of pore formation, pore expansion, and syncytiogenesis.
Figures
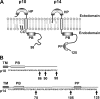
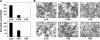
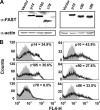
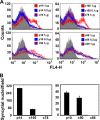
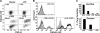
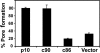
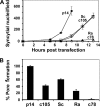
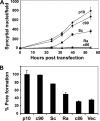
Similar articles
-
Efficient reovirus- and measles virus-mediated pore expansion during syncytium formation is dependent on annexin A1 and intracellular calcium.J Virol. 2014 Jun;88(11):6137-47. doi: 10.1128/JVI.00121-14. Epub 2014 Mar 19. J Virol. 2014. PMID: 24648446 Free PMC article.
-
Extensive syncytium formation mediated by the reovirus FAST proteins triggers apoptosis-induced membrane instability.J Virol. 2005 Jul;79(13):8090-100. doi: 10.1128/JVI.79.13.8090-8100.2005. J Virol. 2005. PMID: 15956554 Free PMC article.
-
Polycistronic Genome Segment Evolution and Gain and Loss of FAST Protein Function during Fusogenic Orthoreovirus Speciation.Viruses. 2020 Jun 29;12(7):702. doi: 10.3390/v12070702. Viruses. 2020. PMID: 32610593 Free PMC article.
-
Reovirus FAST proteins: virus-encoded cellular fusogens.Trends Microbiol. 2014 Dec;22(12):715-24. doi: 10.1016/j.tim.2014.08.005. Epub 2014 Sep 19. Trends Microbiol. 2014. PMID: 25245455 Review.
-
Fusogenic Reoviruses and Their Fusion-Associated Small Transmembrane (FAST) Proteins.Annu Rev Virol. 2019 Sep 29;6(1):341-363. doi: 10.1146/annurev-virology-092818-015523. Epub 2019 Jul 5. Annu Rev Virol. 2019. PMID: 31283438 Review.
Cited by
-
Cell-cell membrane fusion induced by p15 fusion-associated small transmembrane (FAST) protein requires a novel fusion peptide motif containing a myristoylated polyproline type II helix.J Biol Chem. 2012 Jan 27;287(5):3403-14. doi: 10.1074/jbc.M111.305268. Epub 2011 Dec 14. J Biol Chem. 2012. PMID: 22170056 Free PMC article.
-
The EFF-1A Cytoplasmic Domain Influences Hypodermal Cell Fusions in C. elegans But Is Not Dependent on 14-3-3 Proteins.PLoS One. 2016 Jan 22;11(1):e0146874. doi: 10.1371/journal.pone.0146874. eCollection 2016. PLoS One. 2016. PMID: 26800457 Free PMC article.
-
Efficient reovirus- and measles virus-mediated pore expansion during syncytium formation is dependent on annexin A1 and intracellular calcium.J Virol. 2014 Jun;88(11):6137-47. doi: 10.1128/JVI.00121-14. Epub 2014 Mar 19. J Virol. 2014. PMID: 24648446 Free PMC article.
-
Isolation and identification of a novel goose-origin reovirus GD218 and its pathogenicity experiments.Front Vet Sci. 2024 Oct 25;11:1423122. doi: 10.3389/fvets.2024.1423122. eCollection 2024. Front Vet Sci. 2024. PMID: 39525643 Free PMC article.
-
Mutations in the cytoplasmic domain of the Newcastle disease virus fusion protein confer hyperfusogenic phenotypes modulating viral replication and pathogenicity.J Virol. 2013 Sep;87(18):10083-93. doi: 10.1128/JVI.01446-13. Epub 2013 Jul 10. J Virol. 2013. PMID: 23843643 Free PMC article.
References
Publication types
MeSH terms
Substances
Grants and funding
LinkOut - more resources
Full Text Sources
Research Materials