Evolution of catalases from bacteria to humans
- PMID: 18498226
- PMCID: PMC2959186
- DOI: 10.1089/ars.2008.2046
Evolution of catalases from bacteria to humans
Abstract
Excessive hydrogen peroxide is harmful for almost all cell components, so its rapid and efficient removal is of essential importance for aerobically living organisms. Conversely, hydrogen peroxide acts as a second messenger in signal-transduction pathways. H(2)O(2) is degraded by peroxidases and catalases, the latter being able both to reduce H(2)O(2) to water and to oxidize it to molecular oxygen. Nature has evolved three protein families that are able to catalyze this dismutation at reasonable rates. Two of the protein families are heme enzymes: typical catalases and catalase-peroxidases. Typical catalases comprise the most abundant group found in Eubacteria, Archaeabacteria, Protista, Fungi, Plantae, and Animalia, whereas catalase-peroxidases are not found in plants and animals and exhibit both catalatic and peroxidatic activities. The third group is a minor bacterial protein family with a dimanganese active site called manganese catalases. Although catalyzing the same reaction (2 H(2)O(2)--> 2 H(2)O+ O(2)), the three groups differ significantly in their overall and active-site architecture and the mechanism of reaction. Here, we present an overview of the distribution, phylogeny, structure, and function of these enzymes. Additionally, we report about their physiologic role, response to oxidative stress, and about diseases related to catalase deficiency in humans.
Figures
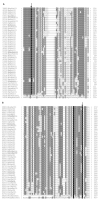
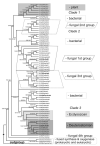
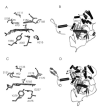
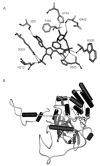
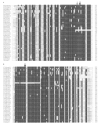
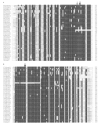
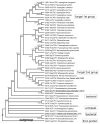
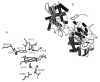
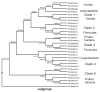
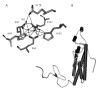
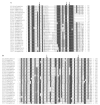
Similar articles
-
Fungal catalases: function, phylogenetic origin and structure.Arch Biochem Biophys. 2012 Sep 15;525(2):170-80. doi: 10.1016/j.abb.2012.05.014. Epub 2012 Jun 12. Arch Biochem Biophys. 2012. PMID: 22698962 Review.
-
Molecular evolution of hydrogen peroxide degrading enzymes.Arch Biochem Biophys. 2012 Sep 15;525(2):131-44. doi: 10.1016/j.abb.2012.01.017. Epub 2012 Feb 7. Arch Biochem Biophys. 2012. PMID: 22330759 Free PMC article. Review.
-
Catalases versus peroxidases: DFT investigation of H₂O₂ oxidation in models systems and implications for heme protein engineering.J Inorg Biochem. 2012 Dec;117:292-7. doi: 10.1016/j.jinorgbio.2012.07.002. Epub 2012 Jul 6. J Inorg Biochem. 2012. PMID: 22883961
-
Turning points in the evolution of peroxidase-catalase superfamily: molecular phylogeny of hybrid heme peroxidases.Cell Mol Life Sci. 2014 Dec;71(23):4681-96. doi: 10.1007/s00018-014-1643-y. Epub 2014 May 21. Cell Mol Life Sci. 2014. PMID: 24846396 Free PMC article.
-
Occurrence, phylogeny, structure, and function of catalases and peroxidases in cyanobacteria.J Exp Bot. 2009;60(2):423-40. doi: 10.1093/jxb/ern309. Epub 2009 Jan 6. J Exp Bot. 2009. PMID: 19129167 Review.
Cited by
-
Detection and characterisation of mutations responsible for allele-specific protein thermostabilities at the Mn-superoxide dismutase gene in the deep-sea hydrothermal vent polychaete Alvinella pompejana.J Mol Evol. 2013 May;76(5):295-310. doi: 10.1007/s00239-013-9559-y. Epub 2013 Apr 23. J Mol Evol. 2013. PMID: 23608997
-
Redox control of leukemia: from molecular mechanisms to therapeutic opportunities.Antioxid Redox Signal. 2013 Apr 10;18(11):1349-83. doi: 10.1089/ars.2011.4258. Epub 2012 Sep 28. Antioxid Redox Signal. 2013. PMID: 22900756 Free PMC article. Review.
-
High Enzyme Activity of a Binuclear Nickel Complex Formed with the Binding Loops of the NiSOD Enzyme*.Chemistry. 2020 Dec 15;26(70):16767-16773. doi: 10.1002/chem.202002706. Epub 2020 Nov 9. Chemistry. 2020. PMID: 32744741 Free PMC article.
-
Transcriptional response of Rhodococcus aetherivorans I24 to polychlorinated biphenyl-contaminated sediments.Microb Ecol. 2010 Oct;60(3):505-15. doi: 10.1007/s00248-010-9650-5. Epub 2010 Apr 6. Microb Ecol. 2010. PMID: 20369357
-
Stability and Catalase-Like Activity of a Mononuclear Non-Heme Oxoiron(IV) Complex in Aqueous Solution.Molecules. 2019 Sep 5;24(18):3236. doi: 10.3390/molecules24183236. Molecules. 2019. PMID: 31491998 Free PMC article.
References
-
- Alfonso-Prieto M, Borovik A, Carpena X, Murshudov G, Melik-Adamyan W, Fita I, Rovira C, Loewen PC. The structures and electronic configuration of compound I intermediates of Helicobacter pylori and Penicillium vitale catalases determined by X-ray crystallography and WM/MM density functional theory calculations. J Am Chem Soc. 2007;129:4193–4205. - PubMed
-
- Antonyuk SV, Melik-Adamyan VR, Popov AN, Lamzin VS, Hempstead PD, Harrison PM, Artymiuk PJ, Barynin VV. Three-dimensional structure of the enzyme dimanganese catalase from Thermus thermophilus at 1A resolution. Crystallogr Rep. 2000;45:105–116.
-
- Baker RD, Cook CO, Goodwin DC. Properties of catalase-peroxidase lacking its C-terminal domain. Biochem Biophys Res Commun. 2004;320:833–839. - PubMed
-
- Barynin VV, Whittaker MM, Antonyuk SV, Lamzin VS, Harrison PM, Artymiuk PJ, Whittaker JW. Crystal structure of manganese catalase from Lactobacillus plantarum. structure. 2001;9:725–738. - PubMed
Publication types
MeSH terms
Substances
Grants and funding
LinkOut - more resources
Full Text Sources
Other Literature Sources
Molecular Biology Databases