Replication fork regression in vitro by the Werner syndrome protein (WRN): holliday junction formation, the effect of leading arm structure and a potential role for WRN exonuclease activity
- PMID: 17717003
- PMCID: PMC2034489
- DOI: 10.1093/nar/gkm561
Replication fork regression in vitro by the Werner syndrome protein (WRN): holliday junction formation, the effect of leading arm structure and a potential role for WRN exonuclease activity
Abstract
The premature aging and cancer-prone disease Werner syndrome stems from loss of WRN protein function. WRN deficiency causes replication abnormalities, sensitivity to certain genotoxic agents, genomic instability and early replicative senescence in primary fibroblasts. As a RecQ helicase family member, WRN is a DNA-dependent ATPase and unwinding enzyme, but also possesses strand annealing and exonuclease activities. RecQ helicases are postulated to participate in pathways responding to replication blockage, pathways possibly initiated by fork regression. In this study, a series of model replication fork substrates were used to examine the fork regression capability of WRN. Our results demonstrate that WRN catalyzes fork regression and Holliday junction formation. This process is an ATP-dependent reaction that is particularly efficient on forks containing single-stranded gaps of at least 11-13 nt on the leading arm at the fork junction. Importantly, WRN exonuclease activity, by digesting the leading daughter strand, enhances regression of forks with smaller gaps on the leading arm, thus creating an optimal structure for regression. Our results suggest that the multiple activities of WRN cooperate to promote replication fork regression. These findings, along with the established cellular consequences of WRN deficiency, strongly support a role for WRN in regression of blocked replication forks.
Figures
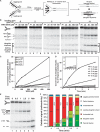
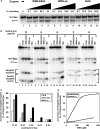
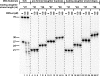
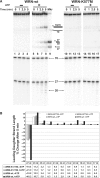
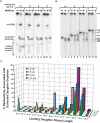
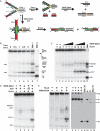
Similar articles
-
Roles of the Werner syndrome RecQ helicase in DNA replication.DNA Repair (Amst). 2008 Nov 1;7(11):1776-86. doi: 10.1016/j.dnarep.2008.07.017. Epub 2008 Sep 6. DNA Repair (Amst). 2008. PMID: 18722555 Free PMC article. Review.
-
Molecular cooperation between the Werner syndrome protein and replication protein A in relation to replication fork blockage.J Biol Chem. 2011 Feb 4;286(5):3497-508. doi: 10.1074/jbc.M110.105411. Epub 2010 Nov 24. J Biol Chem. 2011. PMID: 21107010 Free PMC article.
-
The Werner and Bloom syndrome proteins help resolve replication blockage by converting (regressed) holliday junctions to functional replication forks.Biochemistry. 2011 Aug 16;50(32):6774-88. doi: 10.1021/bi2001054. Epub 2011 Jul 21. Biochemistry. 2011. PMID: 21736299 Free PMC article.
-
The Werner and Bloom syndrome proteins catalyze regression of a model replication fork.Biochemistry. 2006 Nov 28;45(47):13939-46. doi: 10.1021/bi0615487. Biochemistry. 2006. PMID: 17115688
-
Functions of RecQ family helicases: possible involvement of Bloom's and Werner's syndrome gene products in guarding genome integrity during DNA replication.J Biochem. 2001 Apr;129(4):501-7. doi: 10.1093/oxfordjournals.jbchem.a002883. J Biochem. 2001. PMID: 11275547 Review.
Cited by
-
New paradigms in the repair of oxidative damage in human genome: mechanisms ensuring repair of mutagenic base lesions during replication and involvement of accessory proteins.Cell Mol Life Sci. 2015 May;72(9):1679-98. doi: 10.1007/s00018-014-1820-z. Epub 2015 Jan 10. Cell Mol Life Sci. 2015. PMID: 25575562 Free PMC article. Review.
-
Replication protein A stimulates the Werner syndrome protein branch migration activity.J Biol Chem. 2009 Dec 11;284(50):34682-91. doi: 10.1074/jbc.M109.049031. Epub 2009 Oct 7. J Biol Chem. 2009. PMID: 19812417 Free PMC article.
-
RECQL5 plays co-operative and complementary roles with WRN syndrome helicase.Nucleic Acids Res. 2013 Jan;41(2):881-99. doi: 10.1093/nar/gks1134. Epub 2012 Nov 23. Nucleic Acids Res. 2013. PMID: 23180761 Free PMC article.
-
Roles of the Werner syndrome RecQ helicase in DNA replication.DNA Repair (Amst). 2008 Nov 1;7(11):1776-86. doi: 10.1016/j.dnarep.2008.07.017. Epub 2008 Sep 6. DNA Repair (Amst). 2008. PMID: 18722555 Free PMC article. Review.
-
Substrate specific stimulation of NEIL1 by WRN but not the other human RecQ helicases.DNA Repair (Amst). 2010 Jun 4;9(6):636-42. doi: 10.1016/j.dnarep.2010.02.012. Epub 2010 Mar 25. DNA Repair (Amst). 2010. PMID: 20346739 Free PMC article.
References
-
- Goto M. Hierarchical deterioration of body systems in Werner's syndrome: implications for normal ageing. Mech. Ageing Dev. 1997;98:239–254. - PubMed
-
- Martin GM, Oshima J. Lessons from human progeroid syndromes. Nature. 2000;408:263–266. - PubMed
-
- Yu CE, Oshima J, Fu YH, Wijsman EM, Hisama F, Alisch R, Matthews S, Nakura J, Miki T, et al. Positional cloning of the Werner's syndrome gene. Science. 1996;272:258–262. - PubMed
-
- Ellis NA, Groden J, Ye TZ, Straughen J, Lennon DJ, Ciocci S, Proytcheva M, German J. The Bloom's syndrome gene product is homologous to RecQ helicases. Cell. 1995;83:655–666. - PubMed
-
- Kitao S, Shimamoto A, Goto M, Miller RW, Smithson WA, Lindor NM, Furuichi Y. Mutations in RECQL4 cause a subset of cases of Rothmund-Thomson syndrome. Nat. Genet. 1999;22:82–84. - PubMed
Publication types
MeSH terms
Substances
Grants and funding
LinkOut - more resources
Full Text Sources
Research Materials