Mechano- and chemosensitivity of rat nodose neurones--selective excitatory effects of prostacyclin
- PMID: 17478531
- PMCID: PMC2075280
- DOI: 10.1113/jphysiol.2007.133330
Mechano- and chemosensitivity of rat nodose neurones--selective excitatory effects of prostacyclin
Abstract
Nodose ganglion sensory neurones exert a significant reflex autonomic influence. We contrasted their mechanosensitivity, excitability and chemosensitivity in response to the stable prostacyclin (PGI2) analogue carbacyclin (cPGI) in culture. Under current clamp conditions we measured changes in membrane potential (DeltamV) and action potential (AP) responses to mechanically induced depolarizations and depolarizing current injections before and after superfusion of cPGI (1 microM and 10 microM). Chemosensitivity was indicated by augmentation of AP firing frequency and increased maximum gain of AP frequency (max. dAP/dDeltamV), during superfusion with cPGI. Results indicate that two groups of neurones, A and B, are mechanosensitive (MS) and one group, C, is mechanoinsensitive (MI). Group A shows modest depolarization without AP generation during mechanical stimulation, and no increase in max. dAP/dDeltamV, despite a marked increase in electrical depolarization with cPGI. Group B shows pronounced mechanical depolarization accompanied by enhanced AP discharge with cPGI, and an increase in max. dAP/dDeltamV. Group C remains MI after cPGI but is more excitable and markedly chemosensitive (CS) with a pronounced enhancement of max. dAP/dDeltamV with cPGI. The effect of cPGI on ionic conductances indicates that it does not sensitize the mechanically gated depolarizing degenerin/epithelial Na+ channels (DEG/ENaC), but it inhibits two voltage-gated K+ currents, Maxi-K and M-current, causing enhanced AP firing frequency and depolarization, respectively. We conclude that MS nodose neurones may be unimodal MS or bimodal MS/CS, and that MI neurones are unimodal CS, and much more CS to cPGI than MS/CS neurones. We suggest that the known excitatory effect of PGI2 on baroreceptor and vagal afferent fibres is mediated by inhibition of voltage-gated K+ channels (Maxi-K and M-current) and not by an effect on mechanically gated DEG/ENaC channels.
Figures
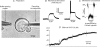
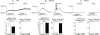
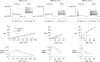
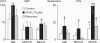
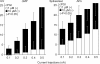
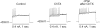
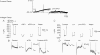
Similar articles
-
The prostacyclin analogue carbacyclin inhibits Ca(2+)-activated K+ current in aortic baroreceptor neurones of rats.J Physiol. 1997 Jun 1;501 ( Pt 2)(Pt 2):275-87. doi: 10.1111/j.1469-7793.1997.275bn.x. J Physiol. 1997. PMID: 9192300 Free PMC article.
-
Inhibitory effects of artemisinin on voltage-gated ion channels in intact nodose ganglion neurones of adult rats.Basic Clin Pharmacol Toxicol. 2007 Apr;100(4):217-24. doi: 10.1111/j.1742-7843.2006.00009.x. Basic Clin Pharmacol Toxicol. 2007. PMID: 17371525
-
Mechanosensory transduction of vagal and baroreceptor afferents revealed by study of isolated nodose neurons in culture.Auton Neurosci. 2002 Jun 28;98(1-2):59-63. doi: 10.1016/s1566-0702(02)00033-4. Auton Neurosci. 2002. PMID: 12144042
-
Differential distribution of voltage-gated channels in myelinated and unmyelinated baroreceptor afferents.Auton Neurosci. 2012 Dec 24;172(1-2):4-12. doi: 10.1016/j.autneu.2012.10.014. Epub 2012 Nov 10. Auton Neurosci. 2012. PMID: 23146622 Review.
-
Mechanosensitive gating of Kv channels.PLoS One. 2015 Feb 13;10(2):e0118335. doi: 10.1371/journal.pone.0118335. eCollection 2015. PLoS One. 2015. PMID: 25680191 Free PMC article. Review.
Cited by
-
Neurobiology of inflammation-associated anorexia.Front Neurosci. 2010 Jan 8;3:59. doi: 10.3389/neuro.23.003.2009. eCollection 2009. Front Neurosci. 2010. PMID: 20582290 Free PMC article.
-
Sensory functions for degenerin/epithelial sodium channels (DEG/ENaC).Adv Genet. 2011;76:1-26. doi: 10.1016/B978-0-12-386481-9.00001-8. Adv Genet. 2011. PMID: 22099690 Free PMC article. Review.
-
Autocrine/paracrine modulation of baroreceptor activity after antidromic stimulation of aortic depressor nerve in vivo.Auton Neurosci. 2014 Feb;180:24-31. doi: 10.1016/j.autneu.2013.10.003. Auton Neurosci. 2014. PMID: 24567955 Free PMC article.
-
The volume-regulated anion channel (LRRC8) in nodose neurons is sensitive to acidic pH.JCI Insight. 2017 Mar 9;2(5):e90632. doi: 10.1172/jci.insight.90632. JCI Insight. 2017. PMID: 28289711 Free PMC article.
-
TRPC5 channels participate in pressure-sensing in aortic baroreceptors.Nat Commun. 2016 Jul 14;7:11947. doi: 10.1038/ncomms11947. Nat Commun. 2016. PMID: 27411851 Free PMC article.
References
-
- Abysique A, Lucchini S, Orsoni P, Mei N, Bouvier M. Effects of alverine citrate on cat intestinal mechanoreceptor responses to chemical and mechanical stimuli. Aliment Pharmacol Ther. 1999;13:561–566. - PubMed
-
- Adaikan PG, Karim SM, Lau LC. Platelet and other effects of carboprostacyclin – a stable prostacyclin analogue. Prostaglandins Med. 1980;5:307–320. - PubMed
-
- Aiken JW, Shebuski RJ. Comparison in anesthetized dogs of the anti-aggregatory and hemodynamic effects of prostacyclin and a chemically stable prostacyclin analog, 6a-carba-PGI2 (carbacyclin) Prostaglandins. 1980;19:629–643. - PubMed
-
- Aiken SP, Zaczek R, Brown BS. Pharmacology of the neurotransmitter release enhancer linopirdine (DuP 996), and insights into its mechanism of action. Adv Pharmacol. 1996;35:349–384. - PubMed
-
- Armour JA, Huang MH, Pelleg A, Sylven C. Responsiveness of in situ canine nodose ganglion afferent neurones to epicardial mechanical or chemical stimuli. Cardiovasc Res. 1994;28:1218–1225. - PubMed
Publication types
MeSH terms
Substances
Grants and funding
LinkOut - more resources
Full Text Sources