Control of single channel conductance in the outer vestibule of the Kv2.1 potassium channel
- PMID: 16880266
- PMCID: PMC2151531
- DOI: 10.1085/jgp.200509465
Control of single channel conductance in the outer vestibule of the Kv2.1 potassium channel
Abstract
Current magnitude in Kv2.1 potassium channels is modulated by external [K+]. In contrast to behavior expected from the change in electrochemical driving force, outward current through Kv2.1 channels becomes larger when extracellular [K+] is increased within the physiological range. The mechanism that underlies this unusual property involves the opening of Kv2.1 channels into one of two different outer vestibule conformations, which are defined by their sensitivity to TEA. Channels that open into a TEA-sensitive conformation generate larger macroscopic currents, whereas channels that open into a TEA-insensitive conformation generate smaller macroscopic currents. At higher [K+], more channels open into the TEA-sensitive conformation. In this manuscript, we examined the mechanism by which the conformational change produced a change in current magnitude. We started by testing the simplest hypothesis: that each pharmacologically defined channel conformation produces a different single channel conductance, one smaller and one larger, and that the [K+]-dependent change in current magnitude reflects the [K+]-dependent change in the percentage of channels that open into each of the two conformations. Using single channel and macroscopic recordings, as well as hidden Markov modeling, we were able to quantitatively account for [K+]-dependent regulation of macroscopic current with this model. Combined with previously published work, these results support a model whereby an outer vestibule lysine interferes with K+ flux through the channel, and that the [K+]-dependent change in orientation of this lysine alters single channel conductance by changing the level of this interference. Moreover, these results provide an experimental example of single channel conductance being modulated at the outer end of the conduction pathway by a mechanism that involves channel activation into open states with different outer vestibule conformations.
Figures
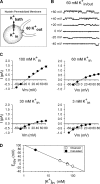
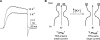
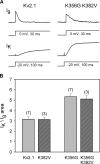
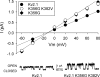
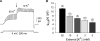
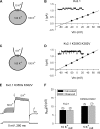
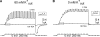
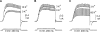
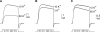
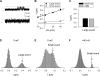
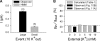
Similar articles
-
Control of outer vestibule dynamics and current magnitude in the Kv2.1 potassium channel.J Gen Physiol. 2002 Nov;120(5):739-55. doi: 10.1085/jgp.20028639. J Gen Physiol. 2002. PMID: 12407083 Free PMC article.
-
Potassium-dependent changes in the conformation of the Kv2.1 potassium channel pore.J Gen Physiol. 1999 Jun;113(6):819-36. doi: 10.1085/jgp.113.6.819. J Gen Physiol. 1999. PMID: 10352033 Free PMC article.
-
Influence of pore residues on permeation properties in the Kv2.1 potassium channel. Evidence for a selective functional interaction of K+ with the outer vestibule.J Gen Physiol. 2003 Feb;121(2):111-24. doi: 10.1085/jgp.20028756. J Gen Physiol. 2003. PMID: 12566539 Free PMC article.
-
Intracellular regions of potassium channels: Kv2.1 and heag.Eur Biophys J. 2009 Mar;38(3):285-92. doi: 10.1007/s00249-008-0354-4. Epub 2008 Jul 8. Eur Biophys J. 2009. PMID: 18607586 Review.
-
How to resolve microsecond current fluctuations in single ion channels: the power of beta distributions.Channels (Austin). 2015;9(5):262-80. doi: 10.1080/19336950.2015.1083660. Channels (Austin). 2015. PMID: 26368656 Free PMC article. Review.
Cited by
-
TRP ion channels: Proteins with conformational flexibility.Channels (Austin). 2019 Dec;13(1):207-226. doi: 10.1080/19336950.2019.1626793. Channels (Austin). 2019. PMID: 31184289 Free PMC article. Review.
-
Distinct mechanisms of inhibition of Kv2 potassium channels by tetraethylammonium and RY785.bioRxiv [Preprint]. 2024 Jul 25:2024.07.25.605170. doi: 10.1101/2024.07.25.605170. bioRxiv. 2024. PMID: 39372771 Free PMC article. Preprint.
-
High spatial density is associated with non-conducting Kv channels from two families.Biophys J. 2022 Mar 1;121(5):755-768. doi: 10.1016/j.bpj.2022.01.021. Epub 2022 Jan 31. Biophys J. 2022. PMID: 35101417 Free PMC article.
-
The tarantula toxin GxTx detains K+ channel gating charges in their resting conformation.J Gen Physiol. 2019 Mar 4;151(3):292-315. doi: 10.1085/jgp.201812213. Epub 2018 Nov 5. J Gen Physiol. 2019. PMID: 30397012 Free PMC article.
-
Localization-dependent activity of the Kv2.1 delayed-rectifier K+ channel.Proc Natl Acad Sci U S A. 2010 Jul 6;107(27):12351-6. doi: 10.1073/pnas.1003028107. Epub 2010 Jun 21. Proc Natl Acad Sci U S A. 2010. PMID: 20566856 Free PMC article.
References
-
- Barry, D.M., J.S. Trimmer, J.P. Merlie, and J.M. Nerbonne. 1995. Differential expression of voltage-gated K+ channel subunits in adult rat heart. Relation to functional K+ channels? Circ. Res. 77:361–369. - PubMed
-
- Bernèche, S., and B. Roux. 2001. Energetics of ion conduction through the K+ channel. Nature. 414:73–77. - PubMed
Publication types
MeSH terms
Substances
Grants and funding
LinkOut - more resources
Full Text Sources
Molecular Biology Databases