Glucose signaling in Saccharomyces cerevisiae
- PMID: 16524925
- PMCID: PMC1393250
- DOI: 10.1128/MMBR.70.1.253-282.2006
Glucose signaling in Saccharomyces cerevisiae
Abstract
Eukaryotic cells possess an exquisitely interwoven and fine-tuned series of signal transduction mechanisms with which to sense and respond to the ubiquitous fermentable carbon source glucose. The budding yeast Saccharomyces cerevisiae has proven to be a fertile model system with which to identify glucose signaling factors, determine the relevant functional and physical interrelationships, and characterize the corresponding metabolic, transcriptomic, and proteomic readouts. The early events in glucose signaling appear to require both extracellular sensing by transmembrane proteins and intracellular sensing by G proteins. Intermediate steps involve cAMP-dependent stimulation of protein kinase A (PKA) as well as one or more redundant PKA-independent pathways. The final steps are mediated by a relatively small collection of transcriptional regulators that collaborate closely to maximize the cellular rates of energy generation and growth. Understanding the nuclear events in this process may necessitate the further elaboration of a new model for eukaryotic gene regulation, called "reverse recruitment." An essential feature of this idea is that fine-structure mapping of nuclear architecture will be required to understand the reception of regulatory signals that emanate from the plasma membrane and cytoplasm. Completion of this task should result in a much improved understanding of eukaryotic growth, differentiation, and carcinogenesis.
Figures
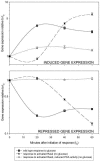
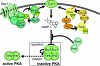
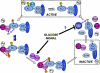
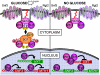
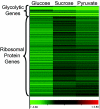
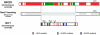
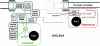
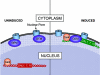
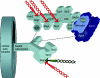
Similar articles
-
Glucose signaling-mediated coordination of cell growth and cell cycle in Saccharomyces cerevisiae.Sensors (Basel). 2010;10(6):6195-240. doi: 10.3390/s100606195. Epub 2010 Jun 21. Sensors (Basel). 2010. PMID: 22219709 Free PMC article. Review.
-
A Saccharomyces cerevisiae G-protein coupled receptor, Gpr1, is specifically required for glucose activation of the cAMP pathway during the transition to growth on glucose.Mol Microbiol. 1999 Jun;32(5):1002-12. doi: 10.1046/j.1365-2958.1999.01413.x. Mol Microbiol. 1999. PMID: 10361302
-
Glucose- and nitrogen sensing and regulatory mechanisms in Saccharomyces cerevisiae.FEMS Yeast Res. 2014 Aug;14(5):683-96. doi: 10.1111/1567-1364.12157. Epub 2014 May 8. FEMS Yeast Res. 2014. PMID: 24738657 Review.
-
Glucose repression in Saccharomyces cerevisiae.FEMS Yeast Res. 2015 Sep;15(6):fov068. doi: 10.1093/femsyr/fov068. Epub 2015 Jul 22. FEMS Yeast Res. 2015. PMID: 26205245 Free PMC article. Review.
-
The molecular chaperone Sse1 and the growth control protein kinase Sch9 collaborate to regulate protein kinase A activity in Saccharomyces cerevisiae.Genetics. 2005 Jul;170(3):1009-21. doi: 10.1534/genetics.105.043109. Epub 2005 May 6. Genetics. 2005. PMID: 15879503 Free PMC article.
Cited by
-
Sda1, a Cys2-His2 zinc finger transcription factor, is involved in polyol metabolism and fumonisin B1 production in Fusarium verticillioides.PLoS One. 2013 Jul 3;8(7):e67656. doi: 10.1371/journal.pone.0067656. Print 2013. PLoS One. 2013. PMID: 23844049 Free PMC article.
-
Developmental Growth Control Exerted via the Protein A Kinase Tpk2 in Ashbya gossypii.Eukaryot Cell. 2015 Jun;14(6):593-601. doi: 10.1128/EC.00045-15. Epub 2015 Apr 10. Eukaryot Cell. 2015. PMID: 25862153 Free PMC article.
-
Glucose signaling-mediated coordination of cell growth and cell cycle in Saccharomyces cerevisiae.Sensors (Basel). 2010;10(6):6195-240. doi: 10.3390/s100606195. Epub 2010 Jun 21. Sensors (Basel). 2010. PMID: 22219709 Free PMC article. Review.
-
Nutrient and Stress Sensing in Pathogenic Yeasts.Front Microbiol. 2019 Mar 8;10:442. doi: 10.3389/fmicb.2019.00442. eCollection 2019. Front Microbiol. 2019. PMID: 30930866 Free PMC article. Review.
-
D-Xylose Sensing in Saccharomyces cerevisiae: Insights from D-Glucose Signaling and Native D-Xylose Utilizers.Int J Mol Sci. 2021 Nov 17;22(22):12410. doi: 10.3390/ijms222212410. Int J Mol Sci. 2021. PMID: 34830296 Free PMC article. Review.
References
-
- Ahn, J. H., S. H. Park, and H. S. Kang. 1995. Inactivation of the UAS1 of STA1 by glucose and STA10 and identification of two loci, SNS1 and MSS1, involved in STA10-dependent repression in Saccharomyces cerevisiae. Mol. Gen. Genet. 246:529-537. - PubMed
-
- Ahuatzi, D., P. Herrero, T. de la Cera, and F. Moreno. 2004. The glucose-regulated nuclear localization of hexokinase 2 in Saccharomyces cerevisiae is Mig1-dependent. J. Biol. Chem. 279:14440-14446. - PubMed
Publication types
MeSH terms
Substances
Grants and funding
LinkOut - more resources
Full Text Sources
Other Literature Sources
Molecular Biology Databases