Nonlinear structured-illumination microscopy: wide-field fluorescence imaging with theoretically unlimited resolution
- PMID: 16141335
- PMCID: PMC1201569
- DOI: 10.1073/pnas.0406877102
Nonlinear structured-illumination microscopy: wide-field fluorescence imaging with theoretically unlimited resolution
Abstract
Contrary to the well known diffraction limit, the fluorescence microscope is in principle capable of unlimited resolution. The necessary elements are spatially structured illumination light and a nonlinear dependence of the fluorescence emission rate on the illumination intensity. As an example of this concept, this article experimentally demonstrates saturated structured-illumination microscopy, a recently proposed method in which the nonlinearity arises from saturation of the excited state. This method can be used in a simple, wide-field (nonscanning) microscope, uses only a single, inexpensive laser, and requires no unusual photophysical properties of the fluorophore. The practical resolving power is determined by the signal-to-noise ratio, which in turn is limited by photobleaching. Experimental results show that a 2D point resolution of <50 nm is possible on sufficiently bright and photostable samples.
Figures
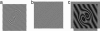
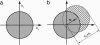
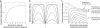
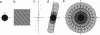
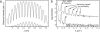
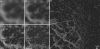
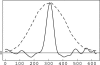
Similar articles
-
Nonlinear structured-illumination microscopy with a photoswitchable protein reveals cellular structures at 50-nm resolution.Proc Natl Acad Sci U S A. 2012 Jan 17;109(3):E135-43. doi: 10.1073/pnas.1107547108. Epub 2011 Dec 12. Proc Natl Acad Sci U S A. 2012. PMID: 22160683 Free PMC article.
-
Practical structured illumination microscopy.Methods Mol Biol. 2015;1251:175-92. doi: 10.1007/978-1-4939-2080-8_10. Methods Mol Biol. 2015. PMID: 25391800
-
The limitations of nonlinear fluorescence effect in super resolution saturated structured illumination microscopy system.J Fluoresc. 2011 May;21(3):1075-82. doi: 10.1007/s10895-010-0780-4. Epub 2010 Dec 30. J Fluoresc. 2011. PMID: 21191638
-
[Comparison and progress review of various super-resolution fluorescence imaging techniques].Se Pu. 2021 Oct;39(10):1055-1064. doi: 10.3724/SP.J.1123.2021.06015. Se Pu. 2021. PMID: 34505427 Free PMC article. Review. Chinese.
-
Recent advances in super-resolution fluorescence imaging and its applications in biology.J Genet Genomics. 2013 Dec 20;40(12):583-95. doi: 10.1016/j.jgg.2013.11.003. Epub 2013 Nov 23. J Genet Genomics. 2013. PMID: 24377865 Review.
Cited by
-
Towards correlative super-resolution fluorescence and electron cryo-microscopy.Biol Cell. 2016 Sep;108(9):245-58. doi: 10.1111/boc.201600008. Epub 2016 Jun 22. Biol Cell. 2016. PMID: 27225383 Free PMC article. Review.
-
Two-dimensional TIRF-SIM-traction force microscopy (2D TIRF-SIM-TFM).Nat Commun. 2021 Apr 12;12(1):2169. doi: 10.1038/s41467-021-22377-9. Nat Commun. 2021. PMID: 33846317 Free PMC article.
-
Super-resolution spectroscopic microscopy via photon localization.Nat Commun. 2016 Jul 25;7:12290. doi: 10.1038/ncomms12290. Nat Commun. 2016. PMID: 27452975 Free PMC article.
-
High-speed videography of transparent media using illumination-based multiplexed schlieren.Sci Rep. 2022 Nov 8;12(1):19018. doi: 10.1038/s41598-022-23198-6. Sci Rep. 2022. PMID: 36347904 Free PMC article.
-
Bleaching/blinking assisted localization microscopy for superresolution imaging using standard fluorescent molecules.Proc Natl Acad Sci U S A. 2011 Dec 27;108(52):21081-6. doi: 10.1073/pnas.1117430109. Epub 2011 Dec 13. Proc Natl Acad Sci U S A. 2011. PMID: 22167805 Free PMC article.
References
Publication types
MeSH terms
Grants and funding
LinkOut - more resources
Full Text Sources
Other Literature Sources
Research Materials