Expansion and compression of a protein folding intermediate by GroEL
- PMID: 15469819
- PMCID: PMC3759401
- DOI: 10.1016/j.molcel.2004.09.003
Expansion and compression of a protein folding intermediate by GroEL
Erratum in
- Mol Cell. 2004 Oct 22;16(2):317
Abstract
The GroEL-GroES chaperonin system is required for the assisted folding of many essential proteins. The precise nature of this assistance remains unclear, however. Here we show that denatured RuBisCO from Rhodospirillum rubrum populates a stable, nonaggregating, and kinetically trapped monomeric state at low temperature. Productive folding of this nonnative intermediate is fully dependent on GroEL, GroES, and ATP. Reactivation of the trapped RuBisCO monomer proceeds through a series of GroEL-induced structural rearrangements, as judged by resonance energy transfer measurements between the amino- and carboxy-terminal domains of RuBisCO. A general mechanism used by GroEL to push large, recalcitrant proteins like RuBisCO toward their native states thus appears to involve two steps: partial unfolding or rearrangement of a nonnative protein upon capture by a GroEL ring, followed by spatial constriction within the GroEL-GroES cavity that favors or enforces compact, folding-competent intermediate states.
Figures
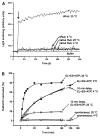
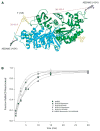
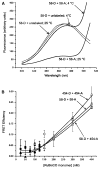
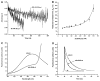
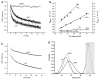
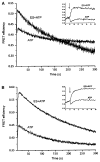
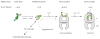
Similar articles
-
GroEL-GroES cycling: ATP and nonnative polypeptide direct alternation of folding-active rings.Cell. 1999 Apr 30;97(3):325-38. doi: 10.1016/s0092-8674(00)80742-4. Cell. 1999. PMID: 10319813
-
No evidence for a forced-unfolding mechanism during ATP/GroES binding to substrate-bound GroEL: no observable protection of metastable Rubisco intermediate or GroEL-bound Rubisco from tritium exchange.FEBS Lett. 2005 Feb 14;579(5):1183-6. doi: 10.1016/j.febslet.2005.01.013. FEBS Lett. 2005. PMID: 15710410
-
Repetitive protein unfolding by the trans ring of the GroEL-GroES chaperonin complex stimulates folding.J Biol Chem. 2013 Oct 25;288(43):30944-55. doi: 10.1074/jbc.M113.480178. Epub 2013 Sep 10. J Biol Chem. 2013. PMID: 24022487 Free PMC article.
-
Reaction Cycle of Chaperonin GroEL via Symmetric "Football" Intermediate.J Mol Biol. 2015 Sep 11;427(18):2912-8. doi: 10.1016/j.jmb.2015.04.007. Epub 2015 Apr 18. J Mol Biol. 2015. PMID: 25900372 Review.
-
Structure and function in GroEL-mediated protein folding.Annu Rev Biochem. 1998;67:581-608. doi: 10.1146/annurev.biochem.67.1.581. Annu Rev Biochem. 1998. PMID: 9759498 Review.
Cited by
-
Cryo-EM structures of GroEL:ES2 with RuBisCO visualize molecular contacts of encapsulated substrates in a double-cage chaperonin.iScience. 2021 Dec 27;25(1):103704. doi: 10.1016/j.isci.2021.103704. eCollection 2022 Jan 21. iScience. 2021. PMID: 35036883 Free PMC article.
-
The C-terminal tails of the bacterial chaperonin GroEL stimulate protein folding by directly altering the conformation of a substrate protein.J Biol Chem. 2014 Aug 15;289(33):23219-23232. doi: 10.1074/jbc.M114.577205. Epub 2014 Jun 25. J Biol Chem. 2014. PMID: 24970895 Free PMC article.
-
ATP-triggered conformational changes delineate substrate-binding and -folding mechanics of the GroEL chaperonin.Cell. 2012 Mar 30;149(1):113-23. doi: 10.1016/j.cell.2012.02.047. Epub 2012 Mar 22. Cell. 2012. PMID: 22445172 Free PMC article.
-
Polypeptide in the chaperonin cage partly protrudes out and then folds inside or escapes outside.EMBO J. 2010 Dec 1;29(23):4008-19. doi: 10.1038/emboj.2010.262. Epub 2010 Oct 19. EMBO J. 2010. PMID: 20959808 Free PMC article.
-
Visualizing GroEL/ES in the act of encapsulating a folding protein.Cell. 2013 Jun 6;153(6):1354-65. doi: 10.1016/j.cell.2013.04.052. Cell. 2013. PMID: 23746846 Free PMC article.
References
-
- Baumketner A, Jewett A, Shea JE. Effects of confinement in chaperonin assisted protein folding: rate enhancement by decreasing the roughness of the folding energy landscape. J Mol Biol. 2003;332:701–713. - PubMed
-
- Bhutani N, Udgaonkar JB. A thermodynamic coupling mechanism can explain the GroEL-mediated acceleration of the folding of barstar. J Mol Biol. 2000;297:1037–1044. - PubMed
-
- Braig K, Otwinowski Z, Hegde R, Boisvert DC, Joachimiak A, Horwich AL, Sigler PB. The crystal structure of the bacterial chaperonin GroEL at 2.8 A. Nature. 1994;371:578–586. - PubMed
-
- Brinker A, Pfeifer G, Kerner MJ, Naylor DJ, Hartl FU, Hayer-Hartl M. Dual function of protein confinement in chaperonin-assisted protein folding. Cell. 2001;107:223–233. - PubMed
Publication types
MeSH terms
Substances
Grants and funding
LinkOut - more resources
Full Text Sources
Molecular Biology Databases
Research Materials