Effects of wortmannin and latrunculin A on slow endocytosis at the frog neuromuscular junction
- PMID: 15004214
- PMCID: PMC1665054
- DOI: 10.1113/jphysiol.2004.062158
Effects of wortmannin and latrunculin A on slow endocytosis at the frog neuromuscular junction
Abstract
Phosphoinositides are key regulators of synaptic vesicle cycling and endocytic traffic; the actin cytoskeleton also seems to be involved in modulating these processes. We investigated the effects of perturbing phosphoinositide signalling and actin dynamics on vesicle cycling in frog motor nerve terminals, using fluorescence and electron microscopy, and electrophysiology. Antibody staining for beta-actin revealed that actin surrounds but does not overlap with synaptic vesicle clusters. Latrunculin A, which disrupts actin filaments by binding actin monomers, and wortmannin, an inhibitor of phosphatidyl inositol-3-kinase (PI3-kinase), each disrupted the pattern of presynaptic actin staining, but not vesicle clusters in resting terminals. Latrunculin A, but not wortmannin, also reduced vesicle mobilization and exocytosis. Both drugs inhibited the stimulation-induced uptake of the styryl dye FM1-43 and blocked vesicle reformation from internalized membrane objects after tetanic stimulation. These results are consistent with a role of PI3-kinase and the actin cytoskeleton in the slow pathway of vesicle endocytosis, used primarily by reserve pool vesicles.
Figures
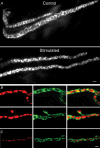
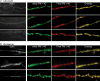
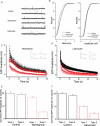
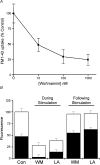
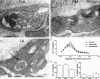
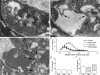
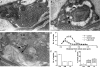
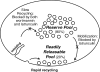
Similar articles
-
Effects of 2-(4-morpholinyl)-8-phenyl-4H-1-benzopyran-4-one on synaptic vesicle cycling at the frog neuromuscular junction.J Neurosci. 2002 Dec 15;22(24):10680-9. doi: 10.1523/JNEUROSCI.22-24-10680.2002. J Neurosci. 2002. PMID: 12486161 Free PMC article.
-
Effects of staurosporine on exocytosis and endocytosis at frog motor nerve terminals.J Neurosci. 2001 Feb 1;21(3):782-7. doi: 10.1523/JNEUROSCI.21-03-00782.2001. J Neurosci. 2001. PMID: 11157064 Free PMC article.
-
Dual pools of actin at presynaptic terminals.J Neurophysiol. 2012 Jun;107(12):3479-92. doi: 10.1152/jn.00789.2011. Epub 2012 Mar 28. J Neurophysiol. 2012. PMID: 22457456 Free PMC article.
-
Exocytosis and endocytosis of synaptic vesicles and functional roles of vesicle pools: lessons from the Drosophila neuromuscular junction.Neuroscientist. 2005 Apr;11(2):138-47. doi: 10.1177/1073858404271679. Neuroscientist. 2005. PMID: 15746382 Review.
-
Phosphoinositides, key molecules for regulation of actin cytoskeletal organization and membrane traffic from the plasma membrane.Biochim Biophys Acta. 2001 Oct 31;1533(3):190-206. doi: 10.1016/s1388-1981(01)00165-2. Biochim Biophys Acta. 2001. PMID: 11731330 Review.
Cited by
-
Synapsin regulates vesicle organization and activity-dependent recycling at Drosophila motor boutons.Neuroscience. 2010 Oct 13;170(2):441-52. doi: 10.1016/j.neuroscience.2010.07.021. Epub 2010 Jul 16. Neuroscience. 2010. PMID: 20638447 Free PMC article.
-
Perturbation of syndapin/PACSIN impairs synaptic vesicle recycling evoked by intense stimulation.J Neurosci. 2008 Apr 9;28(15):3925-33. doi: 10.1523/JNEUROSCI.1754-07.2008. J Neurosci. 2008. PMID: 18400891 Free PMC article.
-
Rho GTPase signaling and mDia facilitate endocytosis via presynaptic actin.Elife. 2024 Mar 19;12:RP92755. doi: 10.7554/eLife.92755. Elife. 2024. PMID: 38502163 Free PMC article.
-
Neutralization of Intracellular pH Homeostasis to Inhibit Osteoclasts Based on a Spatiotemporally Selective Delivery System.Nano Lett. 2023 May 24;23(10):4101-4110. doi: 10.1021/acs.nanolett.2c04295. Epub 2023 May 15. Nano Lett. 2023. PMID: 37183806 Free PMC article.
-
Cholesterol and F-actin are required for clustering of recycling synaptic vesicle proteins in the presynaptic plasma membrane.J Physiol. 2014 Feb 15;592(4):621-33. doi: 10.1113/jphysiol.2013.265447. Epub 2013 Dec 2. J Physiol. 2014. PMID: 24297851 Free PMC article.
References
-
- Aravanis AM, Pyle JL, Tsien RW. Single synaptic vesicles fusing transiently and successively without loss of identity. Nature. 2003;423:643–647. - PubMed
-
- Bai J, Tucker WC, Chapman ER. PIP2 increases the speed of response of synaptotagmin and steers its membrane-penetration activity toward the plasma membrane. Nature Struct Mol Biol. 2004;11:36–44. - PubMed
-
- Betz WJ, Bewick GS. Optical analysis of synaptic vesicle recycling at the frog neuromuscular junction. Science. 1992;225:200–203. - PubMed
-
- Brodin L, Low P, Shupliakov O. Sequential steps in clathrin-mediated synaptic vesicle endocytosis. Curr Opin Neurobiol. 2000;10:312–320. - PubMed
Publication types
MeSH terms
Substances
LinkOut - more resources
Full Text Sources