Inducible systemic RNA silencing in Caenorhabditis elegans
- PMID: 12857879
- PMCID: PMC165691
- DOI: 10.1091/mbc.e03-01-0858
Inducible systemic RNA silencing in Caenorhabditis elegans
Abstract
Introduction of double-stranded RNA (dsRNA) can elicit a gene-specific RNA interference response in a variety of organisms and cell types. In many cases, this response has a systemic character in that silencing of gene expression is observed in cells distal from the site of dsRNA delivery. The molecular mechanisms underlying the mobile nature of RNA silencing are unknown. For example, although cellular entry of dsRNA is possible, cellular exit of dsRNA from normal animal cells has not been directly observed. We provide evidence that transgenic strains of Caenorhabditis elegans transcribing dsRNA from a tissue-specific promoter do not exhibit comprehensive systemic RNA interference phenotypes. In these same animals, modifications of environmental conditions can result in more robust systemic RNA silencing. Additionally, we find that genetic mutations can influence the systemic character of RNA silencing in C. elegans and can separate mechanisms underlying systemic RNA silencing into tissue-specific components. These data suggest that trafficking of RNA silencing signals in C. elegans is regulated by specific physiological and genetic factors.
Figures
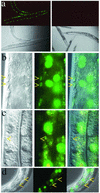
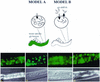
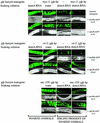
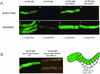
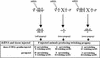
Similar articles
-
Environmental RNA interference.Trends Genet. 2008 Jun;24(6):297-305. doi: 10.1016/j.tig.2008.03.007. Epub 2008 Apr 29. Trends Genet. 2008. PMID: 18450316 Review.
-
Extracellular RNA is transported from one generation to the next in Caenorhabditis elegans.Proc Natl Acad Sci U S A. 2016 Nov 1;113(44):12496-12501. doi: 10.1073/pnas.1608959113. Epub 2016 Oct 17. Proc Natl Acad Sci U S A. 2016. PMID: 27791108 Free PMC article.
-
Gene silencing in Caenorhabditis elegans by transitive RNA interference.RNA. 2003 Jan;9(1):25-32. doi: 10.1261/rna.2650903. RNA. 2003. PMID: 12554873 Free PMC article.
-
SID-1 Domains Important for dsRNA Import in Caenorhabditis elegans.G3 (Bethesda). 2017 Dec 4;7(12):3887-3899. doi: 10.1534/g3.117.300308. G3 (Bethesda). 2017. PMID: 29025917 Free PMC article.
-
How does RNA editing affect dsRNA-mediated gene silencing?Cold Spring Harb Symp Quant Biol. 2006;71:285-92. doi: 10.1101/sqb.2006.71.037. Cold Spring Harb Symp Quant Biol. 2006. PMID: 17381308 Free PMC article. Review.
Cited by
-
ATP-binding cassette transporters are required for efficient RNA interference in Caenorhabditis elegans.Mol Biol Cell. 2006 Aug;17(8):3678-88. doi: 10.1091/mbc.e06-03-0192. Epub 2006 May 24. Mol Biol Cell. 2006. PMID: 16723499 Free PMC article.
-
How will the field of gene therapy survive its success?Bioeng Transl Med. 2018 May 24;3(2):166-177. doi: 10.1002/btm2.10090. eCollection 2018 May. Bioeng Transl Med. 2018. PMID: 30065971 Free PMC article. Review.
-
Exploring systemic RNA interference in insects: a genome-wide survey for RNAi genes in Tribolium.Genome Biol. 2008 Jan 17;9(1):R10. doi: 10.1186/gb-2008-9-1-r10. Genome Biol. 2008. PMID: 18201385 Free PMC article.
-
RNA interference may result in unexpected phenotypes in Caenorhabditis elegans.Nucleic Acids Res. 2019 May 7;47(8):3957-3969. doi: 10.1093/nar/gkz154. Nucleic Acids Res. 2019. PMID: 30838421 Free PMC article.
-
Evidence of a tick RNAi pathway by comparative genomics and reverse genetics screen of targets with known loss-of-function phenotypes in Drosophila.BMC Mol Biol. 2009 Mar 26;10:26. doi: 10.1186/1471-2199-10-26. BMC Mol Biol. 2009. PMID: 19323841 Free PMC article.
References
-
- Alexopoulou, L., Holt, A.C., Medzhitov, R., and Flavell, R.A. (2001). Recognition of double-stranded RNA and activation of NF-kappaB by Toll-like receptor 3. Nature 413, 732-738. - PubMed
-
- Barber, G.N. (2001). Host defense, viruses and apoptosis. Cell Death Differ. 8, 113-126. - PubMed
-
- Baulcombe, D. (2002). RNA silencing. Curr. Biol. 12, R82-R84. - PubMed
-
- Bernstein, E., Caudy, A.A., Hammond, S.M., and Hannon, G.J. (2001). Role for a bidentate ribonuclease in the initiation step of RNA interference. Nature 409, 363-366. - PubMed
Publication types
MeSH terms
Substances
Grants and funding
LinkOut - more resources
Full Text Sources
Other Literature Sources