In vivo whole-cell recording of odor-evoked synaptic transmission in the rat olfactory bulb
- PMID: 12764098
- PMCID: PMC6741073
- DOI: 10.1523/JNEUROSCI.23-10-04108.2003
In vivo whole-cell recording of odor-evoked synaptic transmission in the rat olfactory bulb
Abstract
One of the first steps in the coding of olfactory information is the transformation of synaptic input to action potential firing in mitral and tufted (M/T) cells of the mammalian olfactory bulb. However, little is known regarding the synaptic mechanisms underlying this process in vivo. In this study, we examined odor-evoked response patterns of M/T and granule cells using whole-cell recording in anesthetized, freely breathing rats. We find that odor-evoked excitatory responses in M/T cells typically consist of bursts of action potentials coupled to the approximately 2 Hz respiration rhythm. Odor-evoked, rhythmic M/T cell excitation is reliable during odor presentation (2-4 sec); in contrast, both excitatory responses of granule cells and M/T cell lateral inhibition adapt quickly after the first respiration cycle in the presence of odorants. We also find that the amplitude and initial slope of odor-evoked synaptic excitation play an important role in regulating the timing of M/T cell spikes. Furthermore, differences in odor concentration alter the shape of odor-evoked excitatory synaptic responses, the latency of M/T cell spikes, and the timing of M/T cell lateral inhibition.
Figures
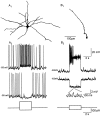
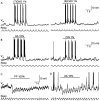
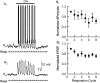
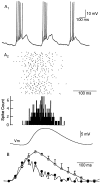
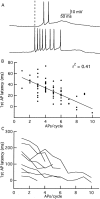
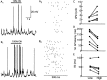
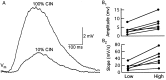
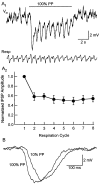
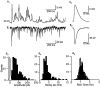
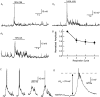
Similar articles
-
Olfactory nerve-evoked, metabotropic glutamate receptor-mediated synaptic responses in rat olfactory bulb mitral cells.J Neurophysiol. 2006 Apr;95(4):2233-41. doi: 10.1152/jn.01150.2005. Epub 2006 Jan 4. J Neurophysiol. 2006. PMID: 16394070 Free PMC article.
-
Direct Recording of Dendrodendritic Excitation in the Olfactory Bulb: Divergent Properties of Local and External Glutamatergic Inputs Govern Synaptic Integration in Granule Cells.J Neurosci. 2017 Dec 6;37(49):11774-11788. doi: 10.1523/JNEUROSCI.2033-17.2017. Epub 2017 Oct 24. J Neurosci. 2017. PMID: 29066560 Free PMC article.
-
Functional Specialization of Interneuron Dendrites: Identification of Action Potential Initiation Zone in Axonless Olfactory Bulb Granule Cells.J Neurosci. 2019 Dec 4;39(49):9674-9688. doi: 10.1523/JNEUROSCI.1763-19.2019. Epub 2019 Oct 29. J Neurosci. 2019. PMID: 31662426 Free PMC article.
-
Electrical signaling in the olfactory bulb.Curr Opin Neurobiol. 2003 Aug;13(4):476-81. doi: 10.1016/s0959-4388(03)00092-8. Curr Opin Neurobiol. 2003. PMID: 12965296 Review.
-
Sensory representations in cerebellar granule cells.Curr Opin Neurobiol. 2009 Aug;19(4):445-51. doi: 10.1016/j.conb.2009.07.003. Epub 2009 Aug 3. Curr Opin Neurobiol. 2009. PMID: 19651506 Review.
Cited by
-
Sparse distributed representation of odors in a large-scale olfactory bulb circuit.PLoS Comput Biol. 2013;9(3):e1003014. doi: 10.1371/journal.pcbi.1003014. Epub 2013 Mar 28. PLoS Comput Biol. 2013. PMID: 23555237 Free PMC article.
-
Linking dynamical and functional properties of intrinsically bursting neurons.J Comput Neurosci. 2013 Oct;35(2):213-30. doi: 10.1007/s10827-013-0449-5. Epub 2013 Apr 11. J Comput Neurosci. 2013. PMID: 23575806
-
Olfactory cortical neurons read out a relative time code in the olfactory bulb.Nat Neurosci. 2013 Jul;16(7):949-57. doi: 10.1038/nn.3407. Epub 2013 May 19. Nat Neurosci. 2013. PMID: 23685720 Free PMC article.
-
Role of intraglomerular circuits in shaping temporally structured responses to naturalistic inhalation-driven sensory input to the olfactory bulb.J Neurophysiol. 2015 May 1;113(9):3112-29. doi: 10.1152/jn.00394.2014. Epub 2015 Feb 25. J Neurophysiol. 2015. PMID: 25717156 Free PMC article.
-
Fast and slow feedforward inhibitory circuits for cortical odor processing.Elife. 2022 Mar 17;11:e73406. doi: 10.7554/eLife.73406. Elife. 2022. PMID: 35297763 Free PMC article.
References
-
- Blanton MG, Lo Turco JJ, Kriegstein AR ( 1989) Whole cell recording from neurons in slices of reptilian and mammalian cerebral cortex. J Neurosci Methods 30: 203–210. - PubMed
-
- Buck L, Axel R ( 1991) A novel multigene family may encode odorant receptors: a molecular basis for odor recognition. Cell 65: 175–187. - PubMed
-
- Chaput M, Holley A ( 1980) Single unit responses of olfactory bulb neurones to odour presentation in awake rabbits. J Physiol (Paris) 76: 551–558. - PubMed
-
- Chaput MA ( 2000) EOG responses in anesthetized freely breathing rats. Chem Senses 25: 695–701. - PubMed
Publication types
MeSH terms
Grants and funding
LinkOut - more resources
Full Text Sources