A novel regulatory element determines the timing of Mos mRNA translation during Xenopus oocyte maturation
- PMID: 12032092
- PMCID: PMC125381
- DOI: 10.1093/emboj/21.11.2798
A novel regulatory element determines the timing of Mos mRNA translation during Xenopus oocyte maturation
Abstract
Progression through vertebrate oocyte maturation requires that pre-existing, maternally derived mRNAs be translated in a strict temporal order. The mechanism that controls the timing of oocyte mRNA translation is unknown. In this study we show that the early translational induction of the mRNA encoding the Mos proto-oncogene is mediated through a novel regulatory element within the 3' untranslated region of the Mos mRNA. This novel element is responsive to the MAP kinase signaling pathway and is distinct from the late acting, cdc2-responsive, cytoplasmic polyadenylation element. Our findings suggest that the timing of maternal mRNA translation is controlled through signal transduction pathways targeting distinct 3' UTR mRNA elements.
Figures
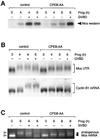
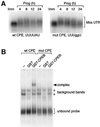
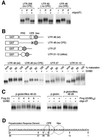
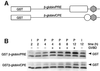
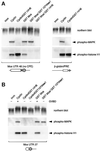
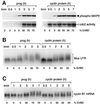
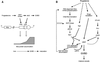
Similar articles
-
Cytoplasmic polyadenylation element (CPE)- and CPE-binding protein (CPEB)-independent mechanisms regulate early class maternal mRNA translational activation in Xenopus oocytes.J Biol Chem. 2004 Apr 23;279(17):17650-9. doi: 10.1074/jbc.M313837200. Epub 2004 Jan 29. J Biol Chem. 2004. PMID: 14752101 Free PMC article.
-
CPEB controls the cytoplasmic polyadenylation of cyclin, Cdk2 and c-mos mRNAs and is necessary for oocyte maturation in Xenopus.EMBO J. 1996 May 15;15(10):2582-92. EMBO J. 1996. PMID: 8665866 Free PMC article.
-
The Mos pathway regulates cytoplasmic polyadenylation in Xenopus oocytes.Mol Cell Biol. 1997 Nov;17(11):6419-26. doi: 10.1128/MCB.17.11.6419. Mol Cell Biol. 1997. PMID: 9343404 Free PMC article.
-
Synthesis and function of Mos: the control switch of vertebrate oocyte meiosis.Bioessays. 1997 Jan;19(1):23-8. doi: 10.1002/bies.950190106. Bioessays. 1997. PMID: 9008414 Review.
-
Unmasking the role of the 3' UTR in the cytoplasmic polyadenylation and translational regulation of maternal mRNAs.Bioessays. 1994 Aug;16(8):533-5. doi: 10.1002/bies.950160804. Bioessays. 1994. PMID: 8086000 Review.
Cited by
-
Developmental timing of mRNA translation--integration of distinct regulatory elements.Mol Reprod Dev. 2010 Aug;77(8):662-9. doi: 10.1002/mrd.21191. Mol Reprod Dev. 2010. PMID: 20652998 Free PMC article. Review.
-
Musashi protein-directed translational activation of target mRNAs is mediated by the poly(A) polymerase, germ line development defective-2.J Biol Chem. 2014 May 16;289(20):14239-51. doi: 10.1074/jbc.M114.548271. Epub 2014 Mar 18. J Biol Chem. 2014. PMID: 24644291 Free PMC article.
-
Autoregulation of Musashi1 mRNA translation during Xenopus oocyte maturation.Mol Reprod Dev. 2012 Aug;79(8):553-63. doi: 10.1002/mrd.22060. Epub 2012 Jul 9. Mol Reprod Dev. 2012. PMID: 22730340 Free PMC article.
-
Cytoplasmic polyadenylation element (CPE)- and CPE-binding protein (CPEB)-independent mechanisms regulate early class maternal mRNA translational activation in Xenopus oocytes.J Biol Chem. 2004 Apr 23;279(17):17650-9. doi: 10.1074/jbc.M313837200. Epub 2004 Jan 29. J Biol Chem. 2004. PMID: 14752101 Free PMC article.
-
CPEB4 is regulated during cell cycle by ERK2/Cdk1-mediated phosphorylation and its assembly into liquid-like droplets.Elife. 2016 Nov 1;5:e19298. doi: 10.7554/eLife.19298. Elife. 2016. PMID: 27802129 Free PMC article.
References
-
- Abrieu A., Brassac,T., Galas,S., Fisher,D., Labbe,J.C. and Doree,M. (1998) The Polo-like kinase Plx1 is a component of the MPF amplification loop at the G2/M-phase transition of the cell cycle in Xenopus eggs. J. Cell Sci., 111, 1751–1757. - PubMed
-
- Barkoff A.F., Dickson,K.S., Gray,N.K. and Wickens,M. (2000) Translational control of cyclin B1 mRNA during meiotic maturation: coordinated repression and cytoplasmic polyadenylation. Dev. Biol., 220, 97–109. - PubMed
-
- Charlesworth A., Welk,J. and MacNicol,A. (2000) The temporal control of Wee1 mRNA translation during Xenopus oocyte maturation is regulated by cytoplasmic polyadenylation elements within the 3′ untranslated region. Dev. Biol., 227, 706–719. - PubMed
Publication types
MeSH terms
Substances
Grants and funding
LinkOut - more resources
Full Text Sources
Other Literature Sources
Miscellaneous