Efficient polyadenylation of Rous sarcoma virus RNA requires the negative regulator of splicing element
- PMID: 11809895
- PMCID: PMC100303
- DOI: 10.1093/nar/30.3.810
Efficient polyadenylation of Rous sarcoma virus RNA requires the negative regulator of splicing element
Abstract
Rous sarcoma virus pre-mRNA contains an element known as the negative regulator of splicing (NRS) that acts to inhibit viral RNA splicing. The NRS binds serine/arginine-rich (SR) proteins, hnRNP H and the U1/U11 snRNPs, and appears to inhibit splicing by acting as a decoy 5' splice site. Deletions within the gag gene that encompass the NRS also lead to increased read-through past the viral polyadenylation site, suggesting a role for the NRS in promoting polyadenylation. Using NRS-specific deletions and mutations, we show here that a polyadenylation stimulatory activity maps directly to the NRS and is most likely dependent upon SR proteins and U1 and/or U11 snRNP. hnRNP H does not appear to mediate splicing control or stimulate RSV polyadenylation, since viral RNAs containing hnRNP H-specific mutations were spliced and polyadenylated normally. However, the ability of hnRNP H mutations to suppress the read-through caused by an SR protein mutation suggests the potential for hnRNP H to antagonize polyadenylation. Interestingly, disruption of splicing control closely correlated with increased read-through, indicating that a functional NRS is necessary for efficient RSV polyadenylation rather than binding of an individual factor. We propose a model in which the NRS serves to enhance polyadenylation of RSV unspliced RNA in a process analogous to the stimulation of cellular pre-mRNA polyadenylation by splicing complexes.
Figures
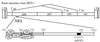
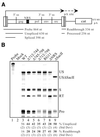
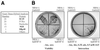
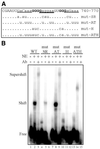
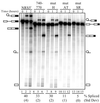
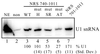
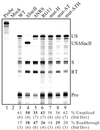
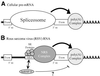
Similar articles
-
A cellular protein, hnRNP H, binds to the negative regulator of splicing element from Rous sarcoma virus.J Biol Chem. 2000 Oct 13;275(41):32371-8. doi: 10.1074/jbc.M005000200. J Biol Chem. 2000. PMID: 10934202
-
Heterogeneous nuclear ribonucleoprotein H is required for optimal U11 small nuclear ribonucleoprotein binding to a retroviral RNA-processing control element: implications for U12-dependent RNA splicing.J Biol Chem. 2006 Feb 3;281(5):2478-88. doi: 10.1074/jbc.M511215200. Epub 2005 Nov 23. J Biol Chem. 2006. PMID: 16308319
-
The negative regulator of splicing element of Rous sarcoma virus promotes polyadenylation.J Virol. 2006 Oct;80(19):9634-40. doi: 10.1128/JVI.00845-06. J Virol. 2006. PMID: 16973567 Free PMC article.
-
U1 snRNP telescripting: molecular mechanisms and beyond.RNA Biol. 2021 Nov;18(11):1512-1523. doi: 10.1080/15476286.2021.1872963. Epub 2021 Jan 15. RNA Biol. 2021. PMID: 33416026 Free PMC article. Review.
-
hnRNP proteins and splicing control.Adv Exp Med Biol. 2007;623:123-47. doi: 10.1007/978-0-387-77374-2_8. Adv Exp Med Biol. 2007. PMID: 18380344 Review.
Cited by
-
Stress proteins: the biological functions in virus infection, present and challenges for target-based antiviral drug development.Signal Transduct Target Ther. 2020 Jul 13;5(1):125. doi: 10.1038/s41392-020-00233-4. Signal Transduct Target Ther. 2020. PMID: 32661235 Free PMC article. Review.
-
SR protein-mediated inhibition of CFTR exon 9 inclusion: molecular characterization of the intronic splicing silencer.Nucleic Acids Res. 2007;35(13):4359-68. doi: 10.1093/nar/gkm444. Epub 2007 Jun 18. Nucleic Acids Res. 2007. PMID: 17576688 Free PMC article.
-
Serine/arginine-rich proteins contribute to negative regulator of splicing element-stimulated polyadenylation in rous sarcoma virus.J Virol. 2007 Oct;81(20):11208-17. doi: 10.1128/JVI.00919-07. Epub 2007 Aug 1. J Virol. 2007. PMID: 17670832 Free PMC article.
-
Structural and functional analysis of the Rous Sarcoma virus negative regulator of splicing and demonstration of its activation by the 9G8 SR protein.Nucleic Acids Res. 2011 Apr;39(8):3388-403. doi: 10.1093/nar/gkq1114. Epub 2010 Dec 22. Nucleic Acids Res. 2011. PMID: 21183462 Free PMC article.
-
RNA processing control in avian retroviruses.Front Biosci. 2008 May 1;13:3869-83. doi: 10.2741/2975. Front Biosci. 2008. PMID: 18508481 Free PMC article. Review.
References
-
- Moore M.J., Query,C.C. and Sharp,P.A. (1993) In Gesteland,R. and Atkins,J. (eds), The RNA World. Cold Spring Harbor Laboratory Press, Cold Spring Harbor, NY, pp. 303–357.
-
- Coffin J.M. (1996) In Fields,B.N., Knipe,D.M. and Howley,P.M. (eds), Fields Virology, 3rd Edn. Raven Press, New York, NY, pp. 1767–1847.
Publication types
MeSH terms
Substances
Grants and funding
LinkOut - more resources
Full Text Sources
Molecular Biology Databases
Research Materials
Miscellaneous