Functional triads consisting of ryanodine receptors, Ca(2+) channels, and Ca(2+)-activated K(+) channels in bullfrog sympathetic neurons. Plastic modulation of action potential
- PMID: 11055998
- PMCID: PMC2229477
- DOI: 10.1085/jgp.116.5.697
Functional triads consisting of ryanodine receptors, Ca(2+) channels, and Ca(2+)-activated K(+) channels in bullfrog sympathetic neurons. Plastic modulation of action potential
Abstract
Fluorescent ryanodine revealed the distribution of ryanodine receptors in the submembrane cytoplasm (less than a few micrometers) of cultured bullfrog sympathetic ganglion cells. Rises in cytosolic Ca(2+) ([Ca(2+)](i)) elicited by single or repetitive action potentials (APs) propagated at a high speed (150 microm/s) in constant amplitude and rate of rise in the cytoplasm bearing ryanodine receptors, and then in the slower, waning manner in the deeper region. Ryanodine (10 microM), a ryanodine receptor blocker (and/or a half opener), or thapsigargin (1-2 microM), a Ca(2+)-pump blocker, or omega-conotoxin GVIA (omega-CgTx, 1 microM), a N-type Ca(2+) channel blocker, blocked the fast propagation, but did not affect the slower spread. Ca(2+) entry thus triggered the regenerative activation of Ca(2+)-induced Ca(2+) release (CICR) in the submembrane region, followed by buffered Ca(2+) diffusion in the deeper cytoplasm. Computer simulation assuming Ca(2+) release in the submembrane region reproduced the Ca(2+) dynamics. Ryanodine or thapsigargin decreased the rate of spike repolarization of an AP to 80%, but not in the presence of iberiotoxin (IbTx, 100 nM), a BK-type Ca(2+)-activated K(+) channel blocker, or omega-CgTx, both of which decreased the rate to 50%. The spike repolarization rate and the amplitude of a single AP-induced rise in [Ca(2+)](i) gradually decreased to a plateau during repetition of APs at 50 Hz, but reduced less in the presence of ryanodine or thapsigargin. The amplitude of each of the [Ca(2+)](i) rise correlated well with the reduction in the IbTx-sensitive component of spike repolarization. The apamin-sensitive SK-type Ca(2+)-activated K(+) current, underlying the afterhyperpolarization of APs, increased during repetitive APs, decayed faster than the accompanying rise in [Ca(2+)](i), and was suppressed by CICR blockers. Thus, ryanodine receptors form a functional triad with N-type Ca(2+) channels and BK channels, and a loose coupling with SK channels in bullfrog sympathetic neurons, plastically modulating AP.
Figures
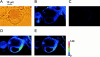
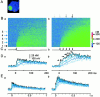
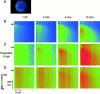
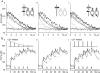
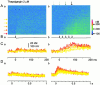
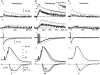
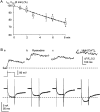
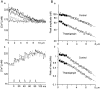
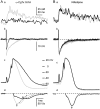
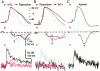
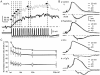
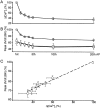
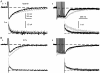
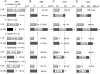
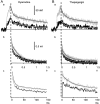
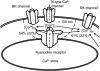
Comment in
-
Functional interactions in Ca(2+) signaling over different time and distance scales.J Gen Physiol. 2000 Nov;116(5):691-6. doi: 10.1085/jgp.116.5.691. J Gen Physiol. 2000. PMID: 11055997 Free PMC article. No abstract available.
Similar articles
-
Spontaneous miniature hyperpolarizations affect threshold for action potential generation in mudpuppy cardiac neurons.J Neurophysiol. 2002 Sep;88(3):1119-27. doi: 10.1152/jn.2002.88.3.1119. J Neurophysiol. 2002. PMID: 12205133
-
Intracellular calcium dynamics in response to action potentials in bullfrog sympathetic ganglion cells.J Physiol. 1992 Dec;458:171-90. doi: 10.1113/jphysiol.1992.sp019412. J Physiol. 1992. PMID: 1302263 Free PMC article.
-
Sources of Ca2+ for different Ca(2+)-activated K+ conductances in neurones of the rat superior cervical ganglion.J Physiol. 1996 Sep 1;495 ( Pt 2)(Pt 2):353-66. doi: 10.1113/jphysiol.1996.sp021599. J Physiol. 1996. PMID: 8887749 Free PMC article.
-
Ca(2+)-induced Ca2+ release in neurones.Jpn J Physiol. 1994;44(6):613-50. doi: 10.2170/jjphysiol.44.613. Jpn J Physiol. 1994. PMID: 7760519 Review. No abstract available.
-
Separation and modulation of calcium currents in bullfrog sympathetic neurons.Can J Physiol Pharmacol. 1992;70 Suppl:S56-63. doi: 10.1139/y92-244. Can J Physiol Pharmacol. 1992. PMID: 1338298 Review.
Cited by
-
Knockout of the BK β4-subunit promotes a functional coupling of BK channels and ryanodine receptors that mediate a fAHP-induced increase in excitability.J Neurophysiol. 2016 Aug 1;116(2):456-65. doi: 10.1152/jn.00857.2015. Epub 2016 May 4. J Neurophysiol. 2016. PMID: 27146987 Free PMC article.
-
Voltage-Induced Ca²⁺ Release in Postganglionic Sympathetic Neurons in Adult Mice.PLoS One. 2016 Feb 9;11(2):e0148962. doi: 10.1371/journal.pone.0148962. eCollection 2016. PLoS One. 2016. PMID: 26859144 Free PMC article.
-
Calcium-induced calcium release and type 3 ryanodine receptors modulate the slow afterhyperpolarising current, sIAHP, and its potentiation in hippocampal pyramidal neurons.PLoS One. 2020 Jun 19;15(6):e0230465. doi: 10.1371/journal.pone.0230465. eCollection 2020. PLoS One. 2020. PMID: 32559219 Free PMC article.
-
Age-dependent changes in Ca2+ homeostasis in peripheral neurones: implications for changes in function.Aging Cell. 2007 Jun;6(3):285-96. doi: 10.1111/j.1474-9726.2007.00298.x. Aging Cell. 2007. PMID: 17517039 Free PMC article. Review.
-
Ca(V)1 and Ca(V)2 channels engage distinct modes of Ca(2+) signaling to control CREB-dependent gene expression.Cell. 2012 May 25;149(5):1112-24. doi: 10.1016/j.cell.2012.03.041. Cell. 2012. PMID: 22632974 Free PMC article.
References
-
- Adams P.R., Constanti A., Brown D.A., Clark R.B. Intracellular Ca2+ activates a fast voltage-sensitive K+ current in vertebrate sympathetic neurones. Nature. 1982;296:746–749. - PubMed
-
- Akita T., Kuba K. Ca2+ release from submembrane and perinuclear Ca2+ stores are involved in action potential-induced Ca2+ transients and modulation of membrane excitability in cultured bullfrog sympathetic neurons Jpn. J. Physiol. 49Suppl.1999. S120a (Abstr.).
-
- Akita T., Kuba K. Ca2+ release from the submembrane and perinuclear Ca2+ stores induced by action potentials and its modulation of the cell membrane excitability in cultured sympathetic neurons Soc. Neurosci. Abstr 25 1999. 1999b (Abstr.).
-
- Allbritton N.L., Meyer T., Stryer L. Range of messenger action of calcium ion and inositol 1,4,5-trisphosphate. Science. 1992;258:1812–1815. - PubMed
Publication types
MeSH terms
Substances
LinkOut - more resources
Full Text Sources
Other Literature Sources
Miscellaneous