Membrane proteins organize a symmetrical virus
- PMID: 11013211
- PMCID: PMC302099
- DOI: 10.1093/emboj/19.19.5081
Membrane proteins organize a symmetrical virus
Abstract
Alphaviruses are enveloped icosahedral viruses that mature by budding at the plasma membrane. According to a prevailing model maturation is driven by binding of membrane protein spikes to a preformed nucleocapsid (NC). The T = 4 geometry of the membrane is thought to be imposed by the NC through one-to-one interactions between spike protomers and capsid proteins (CPs). This model is challenged here by a Semliki Forest virus capsid gene mutant. Its CPs cannot assemble into NCs, or its intermediate structures, due to defective CP-CP interactions. Nevertheless, it can use its horizontal spike-spike interactions on membrane surface and vertical spike-CP interactions to make a particle with correct geometry and protein stoichiometry. Thus, our results highlight the direct role of membrane proteins in organizing the icosahedral conformation of alphaviruses.
Figures
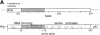
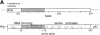
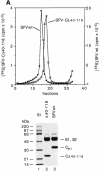
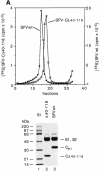
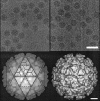
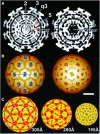
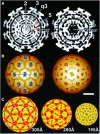
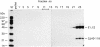
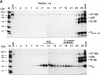
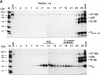
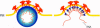
Similar articles
-
An Alphavirus E2 Membrane-Proximal Domain Promotes Envelope Protein Lateral Interactions and Virus Budding.mBio. 2017 Nov 7;8(6):e01564-17. doi: 10.1128/mBio.01564-17. mBio. 2017. PMID: 29114027 Free PMC article.
-
M-X-I motif of semliki forest virus capsid protein affects nucleocapsid assembly.J Virol. 2001 May;75(10):4625-32. doi: 10.1128/JVI.75.10.4625-4632.2001. J Virol. 2001. PMID: 11312332 Free PMC article.
-
A conserved leucine in the cytoplasmic domain of the Semliki Forest virus spike protein is important for budding.Arch Virol. 2000;145(6):1225-30. doi: 10.1007/s007050070121. Arch Virol. 2000. PMID: 10948994
-
Virus maturation by budding.Microbiol Mol Biol Rev. 1998 Dec;62(4):1171-90. doi: 10.1128/MMBR.62.4.1171-1190.1998. Microbiol Mol Biol Rev. 1998. PMID: 9841669 Free PMC article. Review.
-
Incorporation of spike and membrane glycoproteins into coronavirus virions.Viruses. 2015 Apr 3;7(4):1700-25. doi: 10.3390/v7041700. Viruses. 2015. PMID: 25855243 Free PMC article. Review.
Cited by
-
The amino-terminal domain of alphavirus capsid protein is dispensable for viral particle assembly but regulates RNA encapsidation through cooperative functions of its subdomains.J Virol. 2013 Nov;87(22):12003-19. doi: 10.1128/JVI.01960-13. Epub 2013 Sep 4. J Virol. 2013. PMID: 24006447 Free PMC article.
-
Purification and crystallization reveal two types of interactions of the fusion protein homotrimer of Semliki Forest virus.J Virol. 2004 Apr;78(7):3514-23. doi: 10.1128/jvi.78.7.3514-3523.2004. J Virol. 2004. PMID: 15016874 Free PMC article.
-
Cryogenic electron microscopy and tomography reveal imperfect icosahedral symmetry in alphaviruses.PNAS Nexus. 2024 Mar 7;3(3):pgae102. doi: 10.1093/pnasnexus/pgae102. eCollection 2024 Mar. PNAS Nexus. 2024. PMID: 38525304 Free PMC article.
-
Capsid-deficient alphaviruses generate propagative infectious microvesicles at the plasma membrane.Cell Mol Life Sci. 2016 Oct;73(20):3897-916. doi: 10.1007/s00018-016-2230-1. Epub 2016 Apr 27. Cell Mol Life Sci. 2016. PMID: 27117550 Free PMC article.
-
Structural evidence of glycoprotein assembly in cellular membrane compartments prior to Alphavirus budding.J Virol. 2010 Nov;84(21):11145-51. doi: 10.1128/JVI.00036-10. Epub 2010 Aug 25. J Virol. 2010. PMID: 20739526 Free PMC article.
References
-
- Acheson N.H. and Tamm,I. (1967) Replication of Semliki Forest virus: an electron microscopic study. Virology, 32, 128–143. - PubMed
-
- Baker T.S. and Cheng,R.H. (1996) A model-based approach for determining orientations of biological macromolecules imaged by cryoelectron microscopy. J. Struct. Biol., 116, 120–130. - PubMed
-
- Brown D. (1980) The togaviruses: biology, structure and replication. In Schlesinger,R.W. (ed.), The Assembly of Alphaviruses. Academic Press, New York, NY, pp. 473–501.
-
- Cheng R.H. (2000) Visualization of virus–host interactions. In Enquist,B., Johnsson,L. and Nieminen,R.M. (eds), Simulation and Visualization on the Grid. Springer-Verlag, New York, NY, pp. 141–154.
Publication types
MeSH terms
Substances
LinkOut - more resources
Full Text Sources
Other Literature Sources
Miscellaneous