Translational control of viral gene expression in eukaryotes
- PMID: 10839817
- PMCID: PMC98994
- DOI: 10.1128/MMBR.64.2.239-280.2000
Translational control of viral gene expression in eukaryotes
Abstract
As obligate intracellular parasites, viruses rely exclusively on the translational machinery of the host cell for the synthesis of viral proteins. This relationship has imposed numerous challenges on both the infecting virus and the host cell. Importantly, viruses must compete with the endogenous transcripts of the host cell for the translation of viral mRNA. Eukaryotic viruses have thus evolved diverse mechanisms to ensure translational efficiency of viral mRNA above and beyond that of cellular mRNA. Mechanisms that facilitate the efficient and selective translation of viral mRNA may be inherent in the structure of the viral nucleic acid itself and can involve the recruitment and/or modification of specific host factors. These processes serve to redirect the translation apparatus to favor viral transcripts, and they often come at the expense of the host cell. Accordingly, eukaryotic cells have developed antiviral countermeasures to target the translational machinery and disrupt protein synthesis during the course of virus infection. Not to be outdone, many viruses have answered these countermeasures with their own mechanisms to disrupt cellular antiviral pathways, thereby ensuring the uncompromised translation of virion proteins. Here we review the varied and complex translational programs employed by eukaryotic viruses. We discuss how these translational strategies have been incorporated into the virus life cycle and examine how such programming contributes to the pathogenesis of the host cell.
Figures
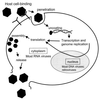
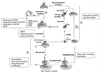
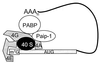
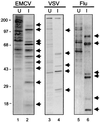
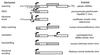
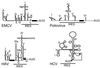
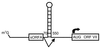
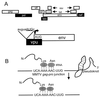
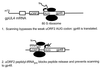
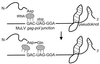
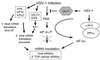
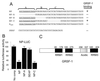
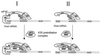
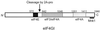
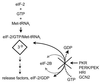
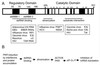
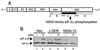
Similar articles
-
Translation initiation and viral tricks.Trends Biochem Sci. 2003 Mar;28(3):130-6. doi: 10.1016/S0968-0004(03)00029-X. Trends Biochem Sci. 2003. PMID: 12633992 Review.
-
Non-Canonical Translation Initiation Mechanisms Employed by Eukaryotic Viral mRNAs.Biochemistry (Mosc). 2021 Sep;86(9):1060-1094. doi: 10.1134/S0006297921090042. Biochemistry (Mosc). 2021. PMID: 34565312 Free PMC article. Review.
-
Tinkering with translation: protein synthesis in virus-infected cells.Cold Spring Harb Perspect Biol. 2013 Jan 1;5(1):a012351. doi: 10.1101/cshperspect.a012351. Cold Spring Harb Perspect Biol. 2013. PMID: 23209131 Free PMC article. Review.
-
Regulation of Tacaribe Mammarenavirus Translation: Positive 5' and Negative 3' Elements and Role of Key Cellular Factors.J Virol. 2017 Jun 26;91(14):e00084-17. doi: 10.1128/JVI.00084-17. Print 2017 Jul 15. J Virol. 2017. PMID: 28468879 Free PMC article.
-
So similar, yet so different: selective translation of capped and polyadenylated viral mRNAs in the influenza virus infected cell.Virus Res. 2011 Mar;156(1-2):1-12. doi: 10.1016/j.virusres.2010.12.016. Epub 2010 Dec 31. Virus Res. 2011. PMID: 21195735 Review.
Cited by
-
Computational based design and tracking of synthetic variants of Porcine circovirus reveal relations between silent genomic information and viral fitness.Sci Rep. 2021 May 19;11(1):10620. doi: 10.1038/s41598-021-89918-6. Sci Rep. 2021. PMID: 34012100 Free PMC article.
-
The dominant-negative inhibition of double-stranded RNA-dependent protein kinase PKR increases the efficacy of Rift Valley fever virus MP-12 vaccine.J Virol. 2012 Jul;86(14):7650-61. doi: 10.1128/JVI.00778-12. Epub 2012 May 9. J Virol. 2012. PMID: 22573861 Free PMC article.
-
Both linear and discontinuous ribosome scanning are used for translation initiation from bicistronic human immunodeficiency virus type 1 env mRNAs.J Virol. 2007 May;81(9):4664-76. doi: 10.1128/JVI.01028-06. Epub 2007 Feb 28. J Virol. 2007. PMID: 17329338 Free PMC article.
-
Effects of influenza A virus NS1 protein on protein expression: the NS1 protein enhances translation and is not required for shutoff of host protein synthesis.J Virol. 2002 Feb;76(3):1206-12. doi: 10.1128/jvi.76.3.1206-1212.2002. J Virol. 2002. PMID: 11773396 Free PMC article.
-
Inhibition of protein kinase R activation and upregulation of GADD34 expression play a synergistic role in facilitating coronavirus replication by maintaining de novo protein synthesis in virus-infected cells.J Virol. 2009 Dec;83(23):12462-72. doi: 10.1128/JVI.01546-09. Epub 2009 Sep 23. J Virol. 2009. PMID: 19776135 Free PMC article.
References
-
- Agol V I. Virus robustness and perseverance. Mol Biol. 1998;32:44–49. - PubMed
-
- Alama A, Barbieri F, Cagnoli M, Schettini G. Antisense oligonucleotides as therapeutic agents. Pharmacol Res. 1997;36:171–178. - PubMed
-
- Ali N, Wang C, Siddiqui A. Translation of hepatitis C virus genome. Princess Takamatsu Symp. 1995;25:99–110. - PubMed
Publication types
MeSH terms
Substances
Grants and funding
LinkOut - more resources
Full Text Sources
Other Literature Sources
Research Materials