RNA sequence and secondary structural determinants in a minimal viral promoter that directs replicase recognition and initiation of genomic plus-strand RNA synthesis
- PMID: 10610788
- PMCID: PMC7172556
- DOI: 10.1006/jmbi.1999.3297
RNA sequence and secondary structural determinants in a minimal viral promoter that directs replicase recognition and initiation of genomic plus-strand RNA synthesis
Abstract
Viral RNA replication provides a useful system to study the structure and function of RNAs and the mechanism of RNA synthesis from RNA templates. Previously we demonstrated that a 27 nt RNA from brome mosaic virus (BMV) can direct correct initiation of genomic plus-strand RNA synthesis by the BMV replicase. In this study, using biochemical, nuclear magnetic resonance, and thermodynamic analyses, we determined that the secondary structure of this 27 nt RNA can be significantly altered and retain the ability to direct RNA synthesis. In contrast, we find that position-specific changes in the RNA sequence will affect replicase recognition, modulate the polymerization process, and contribute to the differential accumulation of viral RNAs. These functional results are in agreement with the phylogenetic analysis of BMV and related viral sequences and suggest that a similar mechanism of RNA synthesis takes place for members of the alphavirus superfamily.
Copyright 1999 Academic Press.
Figures
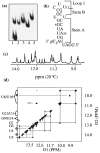
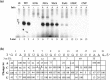
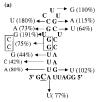
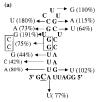
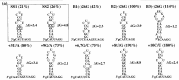
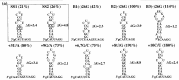
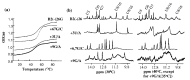
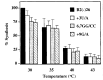
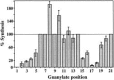
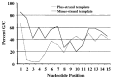
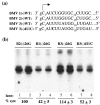
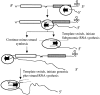
Similar articles
-
Replicase-binding sites on plus- and minus-strand brome mosaic virus RNAs and their roles in RNA replication in plant cells.J Virol. 2004 Dec;78(24):13420-9. doi: 10.1128/JVI.78.24.13420-13429.2004. J Virol. 2004. PMID: 15564452 Free PMC article.
-
Initiation of genomic plus-strand RNA synthesis from DNA and RNA templates by a viral RNA-dependent RNA polymerase.J Virol. 1999 Aug;73(8):6415-23. doi: 10.1128/JVI.73.8.6415-6423.1999. J Virol. 1999. PMID: 10400734 Free PMC article.
-
Recognition of the core RNA promoter for minus-strand RNA synthesis by the replicases of Brome mosaic virus and Cucumber mosaic virus.J Virol. 2000 Nov;74(22):10323-31. doi: 10.1128/jvi.74.22.10323-10331.2000. J Virol. 2000. PMID: 11044076 Free PMC article.
-
Common replication strategies emerging from the study of diverse groups of positive-strand RNA viruses.Arch Virol Suppl. 1994;9:181-94. doi: 10.1007/978-3-7091-9326-6_18. Arch Virol Suppl. 1994. PMID: 8032249 Review.
-
Non-canonical substrates of aminoacyl-tRNA synthetases: the tRNA-like structure of brome mosaic virus genomic RNA.Biochimie. 1993;75(12):1143-57. doi: 10.1016/0300-9084(93)90014-j. Biochimie. 1993. PMID: 8199250 Review.
Cited by
-
Template requirements for RNA synthesis by a recombinant hepatitis C virus RNA-dependent RNA polymerase.J Virol. 2000 Dec;74(23):11121-8. doi: 10.1128/jvi.74.23.11121-11128.2000. J Virol. 2000. PMID: 11070008 Free PMC article.
-
Small noncoding RNA modulates Japanese encephalitis virus replication and translation in trans.Virol J. 2011 Nov 1;8:492. doi: 10.1186/1743-422X-8-492. Virol J. 2011. PMID: 22040380 Free PMC article.
-
In vitro synthesis of minus-strand RNA by an isolated cereal yellow dwarf virus RNA-dependent RNA polymerase requires VPg and a stem-loop structure at the 3' end of the virus RNA.J Virol. 2006 Nov;80(21):10743-51. doi: 10.1128/JVI.01050-06. Epub 2006 Aug 23. J Virol. 2006. PMID: 16928757 Free PMC article.
-
A retroviral RNA kissing complex containing only two G.C base pairs.Proc Natl Acad Sci U S A. 2000 Aug 15;97(17):9396-401. doi: 10.1073/pnas.170283697. Proc Natl Acad Sci U S A. 2000. PMID: 10931958 Free PMC article.
-
Depurination within the intergenic region of Brome mosaic virus RNA3 inhibits viral replication in vitro and in vivo.Nucleic Acids Res. 2008 Dec;36(22):7230-9. doi: 10.1093/nar/gkn896. Epub 2008 Nov 12. Nucleic Acids Res. 2008. PMID: 19004869 Free PMC article.
References
-
- Adkins S., Kao C.C. Subgenomic RNA promoters dictate the mode of recognition by bromoviral RNA-dependent RNA polymerases. Virology. 1998;252:1–8. - PubMed
-
- Ahlquist P. Bromovirus RNA replication and transcription. Curr. Opin. Genet. Dev. 1992;2:71–76. - PubMed
-
- Ahlquist P., Dasgupta R., Kaesberg P. Near identity of 3′ RNA secondary structure in bromoviruses and cucumber mosaic virus. Cell. 1981;23:183–189. - PubMed
Publication types
MeSH terms
Substances
Grants and funding
LinkOut - more resources
Full Text Sources
Research Materials