Quantitative Analysis of NAD Synthesis-Breakdown Fluxes
- PMID: 29685734
- PMCID: PMC5932087
- DOI: 10.1016/j.cmet.2018.03.018
Quantitative Analysis of NAD Synthesis-Breakdown Fluxes
Abstract
The redox cofactor nicotinamide adenine dinucleotide (NAD) plays a central role in metabolism and is a substrate for signaling enzymes including poly-ADP-ribose-polymerases (PARPs) and sirtuins. NAD concentration falls during aging, which has triggered intense interest in strategies to boost NAD levels. A limitation in understanding NAD metabolism has been reliance on concentration measurements. Here, we present isotope-tracer methods for NAD flux quantitation. In cell lines, NAD was made from nicotinamide and consumed largely by PARPs and sirtuins. In vivo, NAD was made from tryptophan selectively in the liver, which then excreted nicotinamide. NAD fluxes varied widely across tissues, with high flux in the small intestine and spleen and low flux in the skeletal muscle. Intravenous administration of nicotinamide riboside or mononucleotide delivered intact molecules to multiple tissues, but the same agents given orally were metabolized to nicotinamide in the liver. Thus, flux analysis can reveal tissue-specific NAD metabolism.
Keywords: NAD; NADH; flux quantification; isotope tracers; mass spectrometry; mononucleotide; niacin; nicotinamide; redox cofactor; riboside.
Copyright © 2018 Elsevier Inc. All rights reserved.
Figures
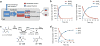
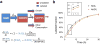
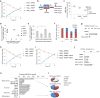
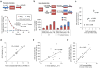
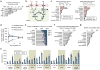
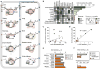
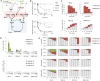
Similar articles
-
Increasing NAD synthesis in muscle via nicotinamide phosphoribosyltransferase is not sufficient to promote oxidative metabolism.J Biol Chem. 2015 Jan 16;290(3):1546-58. doi: 10.1074/jbc.M114.579565. Epub 2014 Nov 19. J Biol Chem. 2015. PMID: 25411251 Free PMC article.
-
NAD+ metabolism in health and disease.Trends Biochem Sci. 2007 Jan;32(1):12-9. doi: 10.1016/j.tibs.2006.11.006. Epub 2006 Dec 11. Trends Biochem Sci. 2007. PMID: 17161604 Review.
-
Implications of NAD+ boosters in translational medicine.Eur J Clin Invest. 2020 Oct;50(10):e13334. doi: 10.1111/eci.13334. Epub 2020 Jul 22. Eur J Clin Invest. 2020. PMID: 32594513 Review.
-
NAD+ and sirtuins in aging and disease.Trends Cell Biol. 2014 Aug;24(8):464-71. doi: 10.1016/j.tcb.2014.04.002. Epub 2014 Apr 29. Trends Cell Biol. 2014. PMID: 24786309 Free PMC article. Review.
-
NRK1 controls nicotinamide mononucleotide and nicotinamide riboside metabolism in mammalian cells.Nat Commun. 2016 Oct 11;7:13103. doi: 10.1038/ncomms13103. Nat Commun. 2016. PMID: 27725675 Free PMC article.
Cited by
-
Tryptophan metabolism and disposition in cancer biology and immunotherapy.Biosci Rep. 2022 Nov 30;42(11):BSR20221682. doi: 10.1042/BSR20221682. Biosci Rep. 2022. PMID: 36286592 Free PMC article.
-
Uncovering the Invisible: Mono-ADP-ribosylation Moved into the Spotlight.Cells. 2021 Mar 19;10(3):680. doi: 10.3390/cells10030680. Cells. 2021. PMID: 33808662 Free PMC article. Review.
-
Synthesis, Optimization, and Structure-Activity Relationships of Nicotinamide Phosphoribosyltransferase (NAMPT) Positive Allosteric Modulators (N-PAMs).J Med Chem. 2023 Dec 28;66(24):16704-16727. doi: 10.1021/acs.jmedchem.3c01406. Epub 2023 Dec 14. J Med Chem. 2023. PMID: 38096366 Free PMC article.
-
Nicotinamide Riboside Augments the Aged Human Skeletal Muscle NAD+ Metabolome and Induces Transcriptomic and Anti-inflammatory Signatures.Cell Rep. 2019 Aug 13;28(7):1717-1728.e6. doi: 10.1016/j.celrep.2019.07.043. Cell Rep. 2019. PMID: 31412242 Free PMC article. Clinical Trial.
-
A duplex structure of SARM1 octamers stabilized by a new inhibitor.Cell Mol Life Sci. 2022 Dec 23;80(1):16. doi: 10.1007/s00018-022-04641-3. Cell Mol Life Sci. 2022. PMID: 36564647 Free PMC article.
References
-
- Buncel E, Lee C. Isotopes in hydrogen transfer processes 1976
MeSH terms
Substances
Grants and funding
LinkOut - more resources
Full Text Sources
Other Literature Sources
Miscellaneous